Organic solar cells (OSCs) have garnered attention in recent years; however, their power conversion efficiencies (PCEs) drop noticeably when green solvents are used. A recent paper published in Nature Energy detailed the use of a guest-assisted assembly strategy to offer a unique approach to creating high-efficiency and large-area OSCs processed from green solvents.
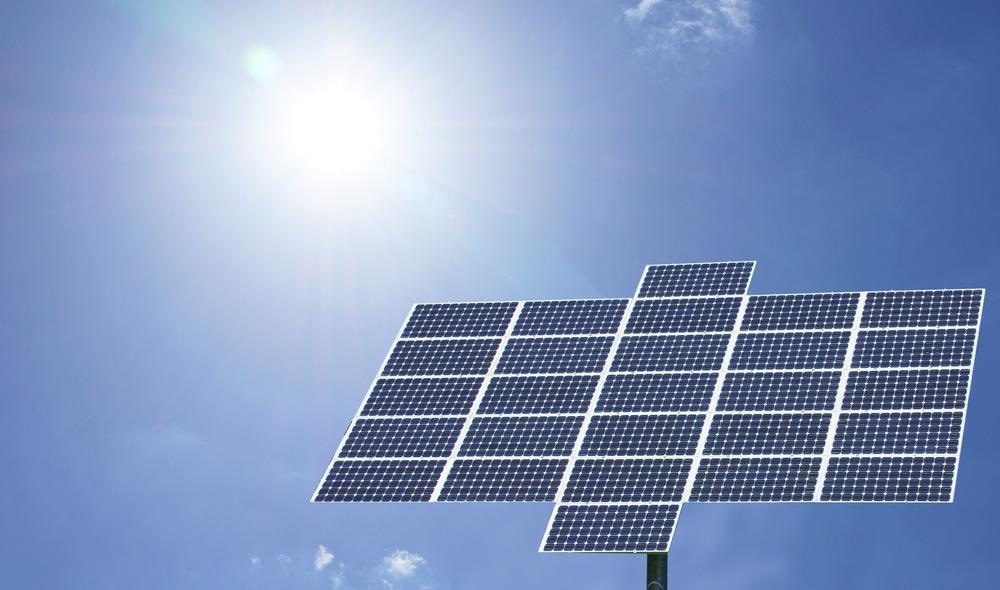
Image Credit: PhotographyByMK/Shutterstock.com
Organic solar cells (OSCs) showed greater advances in the past few years owing to the development of non-fullerene acceptors (NFAs). The superstar NFAs with regards to power conversion efficiencies (PCEs) are dependent on an electron-deficient-core fused ring, called Y-series NFAs. These Y-series NFAs resulted in high PCEs over 18%, making OSCs a topic of commercial relevance.
But these great efficiencies are generally obtained from low-boiling-point and toxic solvents. When high-boiling-point and environmentally friendly solvents are used, there is a drop in the PCEs which might be due to the less solubility of donor and/or acceptor materials. To address this challenge, alkyl side chain engineering for Y-series NFAs was attempted by the researchers.
However, this could not provide the required high efficiency from environmentally friendly solvents. The current study creates a unique guest-assisted assembly strategy to enhance the solubility and molecular packing concurrently in high-boiling-point solvents—in addition to non-halogenated green solvents.
Results
A molecule, BTO (see Figure 1a), having an amphiphilic oligo(ethylene glycol) (OEG) chain is inserted as the guest—which shows better solubility in high-boiling-point solvents and excellent compatibility with the host component (Y6).
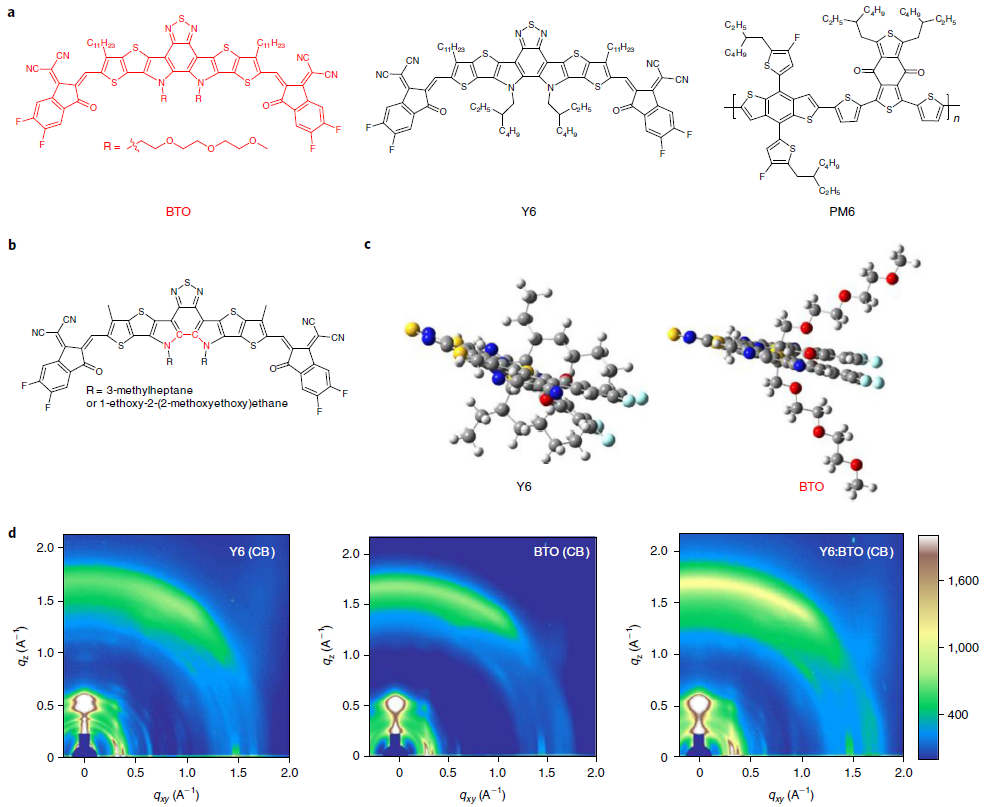
Figure 1. Chemical structures and structural characterization of the acceptors. (a) Chemical structures of BTO, Y6, and PM6. (b) Simplified Y-series molecular structures. (c) The molecular conformation of Y6 and BTO. (d) 2D GIWAXS patterns of pristine Y6, BTO, and Y6:BTO (100:20 weight ratio) processed from CB without thermal annealing (Y6 (CB), BTO (CB), and Y6:BTO (CB)). The color scale bar represents the diffraction intensity in arbitrary units. Image Credit: Chen, et al., 2021
The guest molecule BTO assists in the crystalline organization of Y6. The study also reveals that this approach can be used to blade-coat a large-area OSC module (36 cm2). The guest molecule had the OEG side chains which exhibit conformational flexibility, hydrophilicity, and powerful hydrogen-bonding interactions in conjugated polymers.
The BTO demonstrated remarkable thermal stability with a decomposition temperature of 317 °C. The electrochemical cyclic voltammetry (CV) measurements reveal that BTO has the greatest occupied molecular orbital and least unoccupied molecular orbital energy levels when compared to Y6. The molecular coplanarity and solubility were also increased simultaneously. BTO shows a relatively planar configuration containing a dihedral angle of 4.61°.
The solubility measurements demonstrate that BTO shows greater solubility in both Chloroform (CF) and chlorobenzene (CB). BTO also increased the Y6 solubility in CB. Ultraviolet-visible (UV–vis) absorption spectra reveal that BTO shows a 14 nm red-shifted absorption peak in CB solution, indicating a plane conformation.
It was also noted that the BTO film displays a partly ordered structure driven by OEG side chains. Grazing-incidence wide-angle X-ray scattering (GIWAXS) revealed that the CB-processed Y6 film shows a weak π–π stacking. However, the CB-processed BTO film reveals improved diffraction intensities in both the IP and OOP directions.
A major feature of the BTO molecule is that it can influence the organization of the Y6 molecule in the blend.
The role of the BTO in increasing the crystallinity of Y6 was analyzed by integrating the 2D GIWAXS patterns and was confirmed that BTO induces Y6 to preferentially form a face-on orientation. The researchers later replaced CB with another high-boiling-point solvent—paraxylene (PX)—and found similarly organized structures of Y6.
The ability of the guest molecule BTO to influence the molecular organization of Y6 is preserved in the photovoltaic blend. 2D GIWAXS (see Figure 2a) shows an increased intensity in the IP (100) and OOP (010) of Y6.
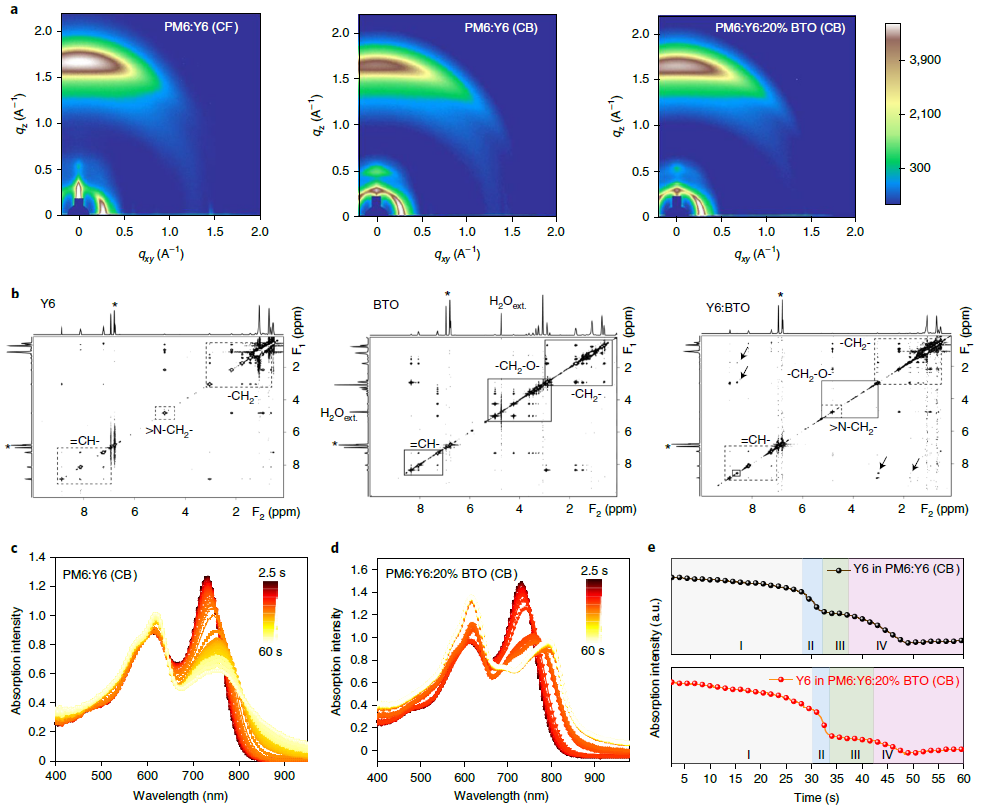
Figure 2. Blend film crystalline properties and intra- and intermolecular interactions between Y6 and BTO. (a) 2D GIWAXS scattering patterns of different blend films. The CF-processed PM6:Y6 film was treated by thermal annealing. (b) Full-range 1H-1H nuclear Overhauser effect spectroscopy (NOESY) 2D NMR spectra recorded with 1-s mixing times of Y6, BTO, and Y6:BTO, where corresponding regions are highlighted using square boxes (dashed for Y6 and solid for BTO). The arrows indicate the intermolecular interaction signals between CH aromatic protons of BTO and protons belonging to the first two -CH2- aliphatic groups of Y6. Peaks with asterisks correspond to solvent CB. (c) In-situ UV–visible absorption of PM6:Y6 (CB) evolution from the solution (2.5 s) to the film (60 s). (d) In-situ UV–visible absorption of PM6:Y6:20% BTO (CB) evolution from solution (2.5 s) to the film (60 s). The color scale bar represents the time. (e) Corresponding changes in the integrated in-situ absorption intensities for PM6 and Y6: quick solvent evaporation stage (light grey area) (I), crystal nucleation stage (light blue area) (II), crystal growth stage (light green area) (III) and film formation stage (light purple area) (IV). Image Credit: Chen, et al., 2021
The researchers also examined the basic reason for the decreased d utilizing 2D NMR and Fourier-transform infrared spectroscopy (FTIR). The results showed strong intermolecular interaction between Y6 and BTO. The strong coplanarity of the BTO molecule aids the ordered arrangement along with the BT units in Y6, increasing the crystallinity.
The FTIR results also demonstrated the same molecular interaction. Molecular interaction between BTO and Y6 on the crystallization mechanism in high-boiling-point solvents was evaluated. The complete mechanism (see Figure 3) of BTO enabling high crystallinity of Y6 from high-boiling-point solvents was obtained.
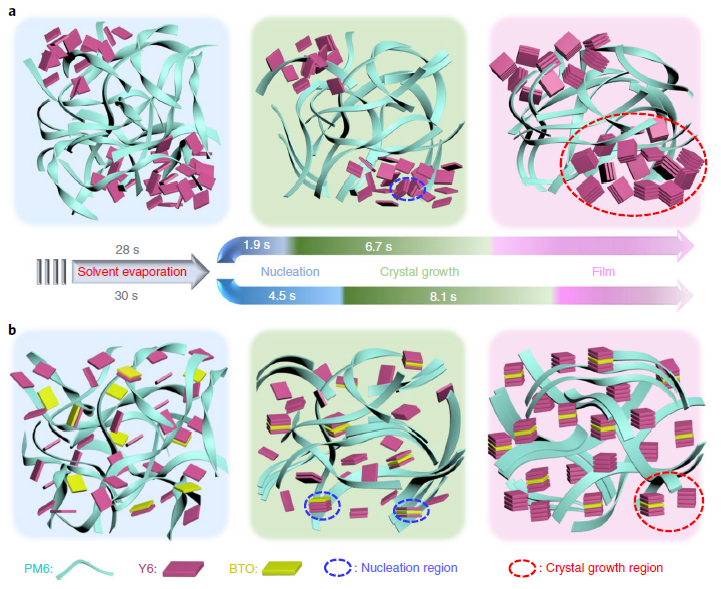
Figure 3. Schematic illustration of BTO-enabling formation of highly crystalline Y6 from high-boiling-point solvents. (a, b) Schematic diagram of the crystallization process for PM6:Y6 (a) and PM6:Y6:20% BTO (b) processed by CB (solvent evaporation stage occurs at 0–28 s for PM6:Y6 and 0–30 s for PM6:Y6:20% BTO). Image Credit: Chen, et al., 2021
The above observations encouraged the researchers to create OSCs made of indium tin oxide which showed a PCE of 16.39% (Table 1). The blend devoid of BTO displayed low PCEs (see Fig. 4a). The PCE was greater than that of the devices where CF was used as the solvent.
Table 1. Photovoltaic parameters of the OSCs on the basis of different active layers under AM 1.5G 100 mW cm−2 illumination Source: Chen, et al., 2021
Active layer |
Voc (V) |
Jsc (mA cm-2) |
FFb |
PCE (%)c |
PM6:Y6 (CF)a |
0.83 |
25.45 |
74.67 |
15.83 (15.69 ± 0.15) |
PM6:Y6 (CB) |
0.82 |
23.19 |
64.29 |
12.17 (12.02 ± 0.16) |
PM6:Y6:20% BTO (CB) |
0.86 |
25.91 |
73.41 |
16.39 (16.20 ± 0.19) |
PM6:Y6:20%
BTO:PC71BM (CB) |
0.86 |
26.66 |
74.26 |
17.08 (16.93 ± 0.15) |
PM6:Y6 (PX) |
0.80 |
22.70 |
61.78 |
11.25 (11.06 ± 0.20) |
PM6:Y6:20% BTO (PX) |
0.85 |
26.32 |
74.32 |
16.59 (16.46 ± 0.13) |
PM6:Y6:20%
BTO:PC71BM (PX) |
0.85 |
27.12 |
75.75 |
17.41 (17.30 ± 0.14) |
aThe OSC based on CF-processed PM6:Y6 active layer with thermal-annealing treatment. bFF represents the fill factor. cAverage and standard deviation data in parentheses are obtained from 20 cells for each condition.
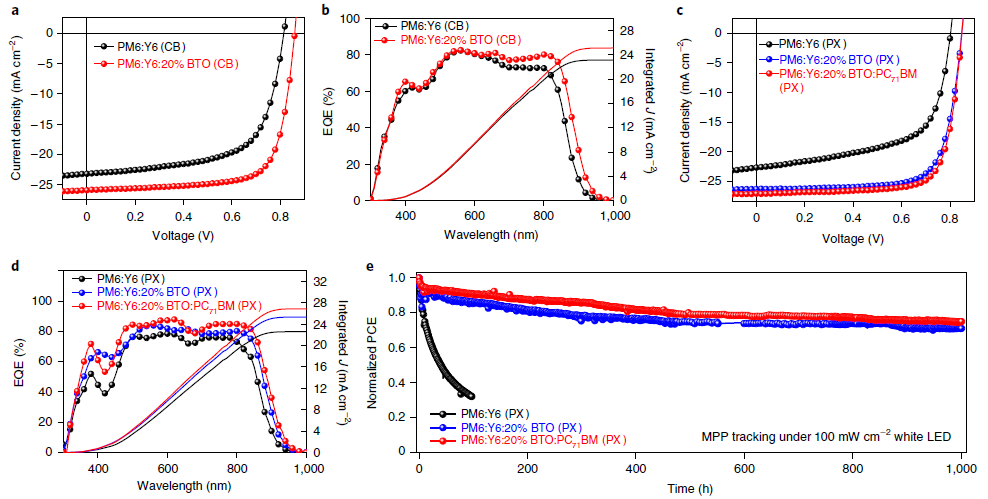
Figure 4. Photovoltaic performance of the devices. a, J–V curves of CB-processed OSCs under the illumination of AM 1.5G 100 mW cm−2. (b) The corresponding EQE spectra. (c) J–V curves of PX-processed OSCs under the illumination of AM 1.5G 100 mW cm−2. (d) The corresponding EQE spectra. (e) MPP stability test of unencapsulated OSCs based on PM6:Y6 (PX), PM6:Y6: 20% BTO (PX), and PM6:Y6: 20% BTO:PC71BM (PX) with initial PCEs of 11.01%, 16.41%, and 17.22%, respectively, stored in N2 atmosphere under the illumination of a 100 mW cm-2 white LED. Image Credit: Chen, et al., 2021
The approach was analyzed for its applicability in non-halogenated high-boiling-point solvents which showed greater efficacy. Along with efficiency, this strategy can also help improve operational stability, owing to the strong intermolecular interaction between BTO and Y6.
Non-halogenated high-boiling-point solvents enhance the development of large-area devices with uniform active layers (see Figure 5a).
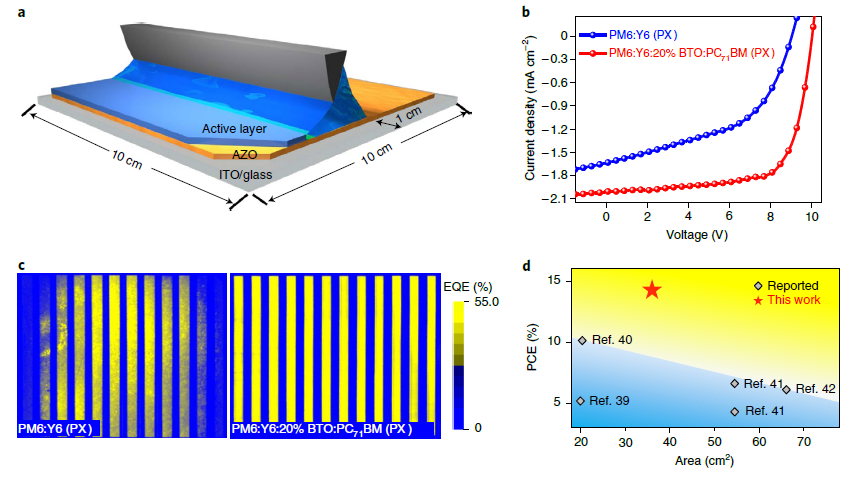
Figure 5. Non-halogenated solvent-processed module and device stability. (a) Schematic illustration of the blade-coating process of PX-processed active layer for large-area OSC modules. (b) The J–V curves of the OSC modules under the illumination of AM 1.5G 100 mW cm−2. (c) The LBIC mapping images of the blade-coated OSCs. The color scale bar represents the intensity of the EQE. d, Distribution of the PCEs of non-halogenated solvent-processed OSC modules in terms of the device area (module area >20 cm2). Image Credit: Zhang, et al., 2017; Liao, et al., 2020; Rasool, et al., 2019; Ibraikulov, et al., 2019
The module’s uniformity was confirmed by light-beam-induced current (LBIC) mapping and the observations indicate a PCE of 14.26%, the highest among OSC modules.
Methodology
The required materials ITO glass, PM6, Y6 and 2-(5,6-difluoro-3-oxo-2,3-dihydro-1H-inden-1-ylidene)malononitrile, CB, PEDOT:PSS were procured. BTP-OEG-CHO was synthesized and purified.
NMR spectra measurements were taken using a Varian Mercury-400 NMR spectrometer. Later high-resolution MALDI-TOF measurements were interpreted followed by Electrical measurements. The external quantum efficiency (EQE) spectra were procured using a QE-R3011 solar cell spectral response measurement system.
Electroluminescence quantum efficiency measurements were evaluated with an electroluminescence measurement system followed by TGA measurements and CV measurements.
The study used an ultraviolet spectrometer to analyze the transmittance and absorption spectra and LBIC mapping measurements were performed with a Professional LBIC. The 2D GIWAXS measurements were performed and the patterns were recorded with a 2D CCD detector.
The characterization took place through the transmission mode. The study also measured FTIR measurements.
Device fabrication was carried out by rinsing ITO-coated glass substrates and spin-coating the AZO layer followed by spin-coating the active layer precursor solutions. Non-halogenated green solvent-processed devices were also developed similarly.
Conclusion
This article details the fabrication of a guest-assisted assembly approach to manipulate the crystallization of photovoltaic materials. The research revealed the fundamental processes linked to guest-assisted assembly mechanism at the molecular level, offering vital information for future investigation of this strategy.
This approach could offer significant contributions to bring OSCs to the next phase and can also be used to maximize other organic electronic devices.
Journal Reference:
Chen, H., Zhang, R., Chen., Zeng, G., Kobera, L., Abbrent, S., Zhang, B., Chen, W., Xu, G., Oh, J., Kang, S.-H., Chen, S., Yang, C., Brus, J., Hou, J., Gao, F., Li, Y., Li., Y. (2021) A guest-assisted molecular-organization approach for >17% efficiency organic solar cells using environmentally friendly solvents. Nature Energy, 6, pp. 1045–1053. Available online: https://www.nature.com/articles/s41560-021-00923-5.
References and Further Reading:
- Zhang, M., et al. (2021) Single-layered organic photovoltaics with double cascading charge transport pathways: 18% efficiencies. Nature Communications, 12, p. 309 doi.org/10.1038/s41467-020-20580-8.
- Liu, Q., et al. (2020) 18% Efficiency organic solar cells. Science Bulletin, 65, pp. 272–275. doi.org/10.1016/j.scib.2020.01.001.
- Li, C., et al. (2021) Non-fullerene acceptors with branched side chains and improved molecular packing to exceed 18% efficiency in organic solar cells. Nature Energy. doi.org/10.1038/s41560-021-00820-x.
- Cui, Y., et al. (2020) Single-junction organic photovoltaic cells with approaching 18% efficiency. Advanced Materials, 32, p. 1908205. doi.org/10.1002/adma.201908205.
- Chen, X., et al. (2021) A unified description of non-radiative voltage losses in organic solar cells. Nature Energy. doi.org/10.1038/s41560-021-00843-4.
- Zhang, H., et al. (2018) Over 14% efficiency in organic solar cells enabled by chlorinated nonfullerene small-molecule acceptors. Advanced Materials, 30, p. 1800613. doi.org/10.1002/adma.201800613.
- Qian, D., et al. (2018) Design rules for minimizing voltage losses in high-efficiency organic solar cells. Nature Materials, 17, pp. 703–709. doi.org/10.1038/s41563-018-0128-z.
- Hou, J., et al. (2018) Organic solar cells based on non-fullerene acceptors. Nature Materials, 17, pp. 119–128. doi.org/10.1038/nmat5063.
- Liu, J., et al. (2016) Fast charge separation in a non-fullerene organic solar cell with a small driving force. Nature Energy, 1, pp. 16089. doi.org/10.1038/nenergy.2016.89.
- Yuan, J., et al. (2019) Single-junction organic solar cell with over 15% efficiency using fused-ring acceptor with electron-deficient core. Joule, 3, pp. 1140–1151. doi.org/10.1016/j.joule.2019.01.004.
- Jiang, K., et al. (2019) Alkyl chain tuning of small molecule acceptors for efficient organic solar cells. Joule, 3, pp. 3020–3033. doi.org/10.1016/j.joule.2019.09.010.
- . Liu, S., et al. (2020) High-efficiency organic solar cells with low non-radiative recombination loss and low energetic disorder. Nature Photonics, 14, pp. 300–305. doi.org/10.1038/s41566-019-0573-5.
- Dong, S., et al. (2020) Single-component non-halogen solvent-processed high-performance organic solar cell module with efficiency over 14%. Joule, 4, pp. 2004–2016. doi.org/10.1016/j.joule.2020.07.028.
- Hong, L. et al. (2019) Eco-compatible solvent-processed organic photovoltaic cells with over 16% efficiency. Advanced Materials, 31, p. 1903441. doi.org/10.1002/adma.201903441.
- . Lin, F., et al. (2020) A non-fullerene acceptor with enhanced intermolecular π-core interaction for high-performance organic solar cells. Journal of American Chemical Society, 142, pp. 15246–15251. doi.org/10.1021/jacs.0c07083.
- Zhu, W., et al. (2020) Crystallography, morphology, electronic structure, and transport in non-fullerene/non-indacenodithienothiophene polymer:Y6 solar cells. Journal of American Chemical Society, 142, pp. 14532–14547. doi.org/10.1021/jacs.0c05560.
- Zhu, L., et al. (2020) Efficient organic solar cell with 16.88% efficiency enabled by refined acceptor crystallization and morphology with improved charge transfer and transport properties. Advanced Energy Materials, 10, p. 1904234. doi.org/10.1002/aenm.201904234.
- Manley, E. F., et al. (2018) In situ analysis of solvent and additive effects on film morphology evolution in spin-cast small-molecule and polymer photovoltaic materials. Advanced Energy Materials, 8, p. 1800611. doi.org/10.1002/aenm.201800611.
- Sun, R., et al. (2020) Achieving eco-compatible organic solar cells with efficiency >16.5% based on an iridium complex-incorporated polymer donor. Solar RRL, 4, p. 2000156. doi.org/10.1002/solr.202000156.
- Torabi, S., et al. (2015) Strategy for enhancing the dielectric constant of organic semiconductors without sacrificing charge carrier mobility and solubility. Advanced Functional Materials, 25, pp. 150–157. doi.org/10.1002/adfm.201402244.
- Chen, X., et al. (2016) Diketopyrrolopyrrole-based conjugated polymers bearing branched oligo(ethylene glycol) side chains for photovoltaic devices. Angewandte Chemie International Edition, 55, pp. 10376–10380. doi.org/10.1002/anie.201602775.
- Meng, B., et al. (2015) Replacing alkyl with oligo(ethylene glycol) as side chains of conjugated polymers for close π-π stacking. Macromolecules, 48, pp. 4357–4363. doi.org/10.1021/acs.macromol.5b00702.
- Meng, B., et al. (2020) Oligo(ethylene glycol) as side chains of conjugated polymers for optoelectronic applications. Polymer Chemistry, 11, pp. 1261–1270. doi.org/10.1039/C9PY01469A.
- Huang, W. Y., et al. (2008) Aggregation and gelation effects on the performance of poly(3-hexylthiophene)/fullerene solar cells. Macromolecules, 41, pp. 7485–7489. doi.org/10.1021/ma801368z.
- Wedler, S., et al. (2020) A role of torsional flexibility in the film formation process in two π-conjugated model oligomers. The Journal of Physical Chemistry Letters, 11, pp. 9379–9386. doi.org/10.1021/acs.jpclett.0c02778.
- Li, S., et al. (2020) Asymmetric electron acceptors for high-efficiency and low-energy-loss organic photovoltaics. Advanced Materials, 32, p. 2001160. doi.org/10.1002/adma.202001160.
- Fan, B., et al. (2018) Fine-tuning of the chemical structure of photoactive materials for highly efficient organic photovoltaics. Nature Energy, 3, pp. 1051–1058. doi.org/10.1038/s41560-018-0263-4.
- Vohra, V., et al. (2015) Efficient inverted polymer solar cells employing favourable molecular orientation. Nature Photonics, 9, pp. 403–408. doi.org/10.1038/nphoton.2015.84.
- Yang, Y. et al. (2015) High-performance multiple-donor bulk heterojunction solar cells. Nature Photonics, 9, p. 190. doi.org/10.1038/nphoton.2015.9.
- Perdigón-Toro, L., et al. (2020) Barrierless free charge generation in the high-performance PM6:Y6 bulk heterojunction non-fullerene solar cell. Advanced Materials, 32, p. 1906763. doi.org/10.1002/adma.201906763.
- Abdelsamie, M., et al. (2014) In situ UV-visible absorption during spin-coating of organic semiconductors: a new probe for organic electronics and photovoltaics. Journal of Materials Chemistry C, 2, pp. 3373–3381. doi.org/10.1039/C3TC32077D.
- Mao, Y., et al. (2019) Evolution of molecular aggregation in bar-coated non-fullerene organic solar cells. Materials Chemistry Frontiers, 3, pp. 1062–1070. doi.org/10.1039/C9QM00078J.
- van Franeker, J. J., et al. (2015) A real-time study of the benefits of co-solvents in polymer solar cell processing. Nature Communications, 6, p. 6229. doi.org/10.1038/ncomms7229.
- Burlingame, Q. et al. (2019) Intrinsically stable organic solar cells under high-intensity illumination. Nature, 573, pp. 394–397. doi.org/10.1038/s41586-019-1544-1.
- Ghasemi, M., et al. (2021) A molecular interaction-diffusion framework for predicting organic solar cell stability. Nature Materials. doi.org/10.1038/s41563-020-00872-6.
- Duan, L., et al. (2019) Comparative analysis of burn-in photo-degradation in non-fullerene COi8DFIC acceptor based high-efficiency ternary organic solar cells. Materials Chemistry Frontiers, 3, pp. 1085–1096. doi.org/10.1039/C9QM00130A.
- Günther, M., et al. (2021) Increasing photostability of inverted nonfullerene organic solar cells by using fullerene derivative additives. ACS Applied Materials & Interfaces, 13, pp. 19072–19084. doi.org/10.1021/acsami.1c00700.
- Park, S., et al. (2020) Progress in materials, solution processes, and long-term stability for large-area organic photovoltaics. Advanced Materials, 32, p. 2002217. doi.org/10.1002/adma.202002217.
- Popović, D., et al. (2017) Preparation of efficient oligomer-based bulk-heterojunction solar cells from eco-friendly solvents. Journal of Material Chemistry C, 5, pp. 9920–9928. doi.org/10.1039/C7TC02131C.
- Pan, F., et al. (2021) High electron mobility fluorinated indacenodithiophene small molecule acceptors for organic solar cells. Chinese Chemical Letters, 32, pp. 1257–1262. doi.org/10.1016/j.cclet.2020.08.051.
- Zhang, W., et al. (2010) Indacenodithiophene semiconducting polymers for high-performance, air-stable transistors. Journal of American Chemical Society, 132, pp. 11437–11439. doi.org/10.1021/ja1049324.
- . Zhang, J., et al. (2017) Enhancing performance of large-area organic solar cells with thick film via ternary strategy. Small, 13, p. 1700388. doi.org/10.1002/smll.201700388.
- Liao, C.-Y., et al. (2020) Processing strategies for an organic photovoltaic module with over 10% efficiency. Joule, 4, pp. 189–206. doi.org/10.1016/j.joule.2019.11.006.
- Rasool, S., et al. (2019) Room temperature processed highly efficient large-area polymer solar cells achieved with molecular engineering of copolymers. Advanced Energy Materials, 9, p. 1900168. doi.org/10.1002/aenm.201900168.
- Ibraikulov, O. A., et al. (2019) ITO-free organic photovoltaic modules based on fluorinated polymers deposited from non-halogenated solution: a major step toward large-scale module production. Solar RRL, 3, p. 1900273. doi.org/10.1002/solr.201900273.