Water covers around 75% of the Earth’s surface. Freshwater, on the other hand, accounts for only about 2.7% of this vast natural resource. Oceans and seas contain almost 97% of the world’s water, which contains salts and minerals that cannot be consumed directly in everyday lives. This article will look at research conducted by Kunjaram, U. P. U et al. published in EcoMat.
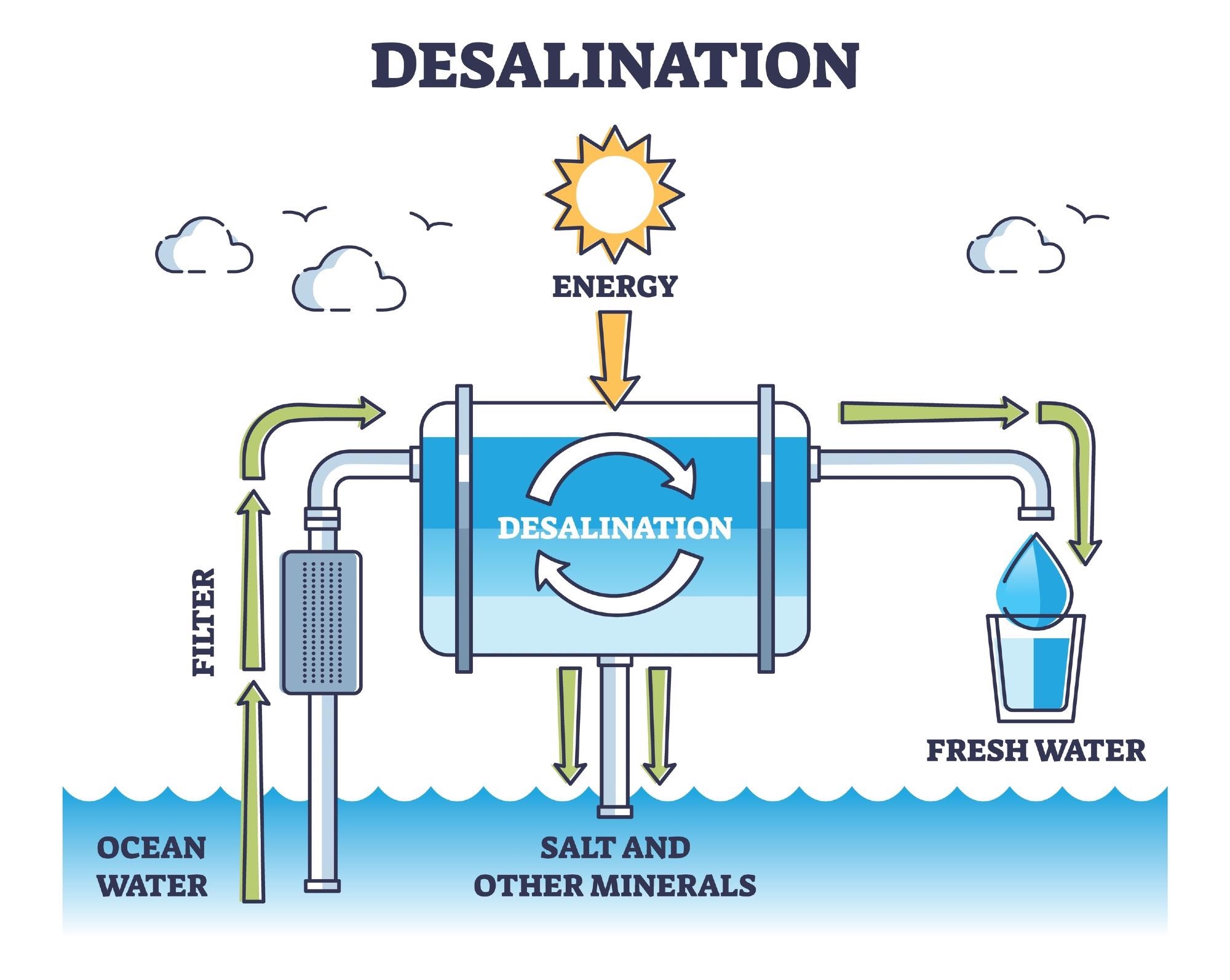
Image Credit: VectorMine/Shutterstock.com
Freshwater shortages are one of humankind’s most important problems. As a result, desalination is the most common method for converting seawater to freshwater for drinking, irrigation, and daily use.
Solar-driven interfacial evaporation has recently attracted a lot of interest because of its potential in terms of water, energy, and ecological sustainability. This method uses solar thermal processes to evaporate water from brines or contaminated water, and it has the potential to achieve zero-liquid-discharge (ZLD) desalination.
To boost overall water production, researchers created better materials, enhanced thermal management strategies and procedures, and cooling technologies throughout the last decade.
When seawater is evaporated using an evaporator under the sun, a salinity gradient forms between the bulk water and the evaporator, enabling salts to diffuse and redistribute. Since solar absorbers are primarily black to allow maximum sunlight uptake, salt accumulation on them is generally regarded as unfavorable. The formation of salt on the absorber obstructs steady vapor generation and degrades the solar thermal efficiency over time.
Figure 1A depicts a typical architecture that uses a controlled water flow to guide salt movement through evaporative substances (e.g., so-called edge preferential salt crystallization).
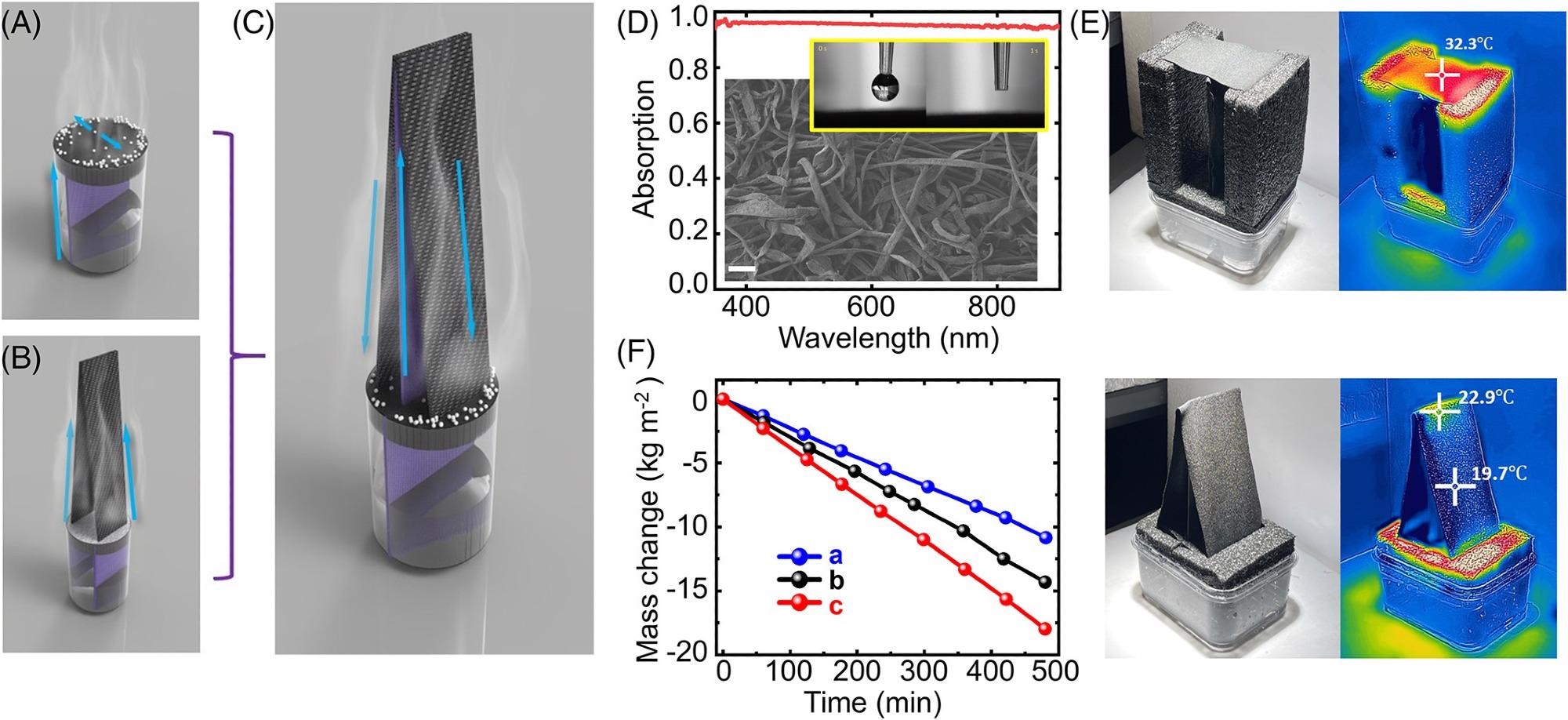
Figure 1. The umbrella architecture for enhanced solar evaporation. (A) Schematic illustration of salt crystallization based on water delivery direction on a flat system. (B) Schematic illustration of the tapered architecture disclosed in reference. (C) Schematic illustration of the umbrella architecture. The water flow directions in these three architectures are indicated by the blue arrows. (D) Optical absorption spectrum of the black surface for water evaporation. The upper inset: the hydrophilic feature of the fabric materials used as the evaporation surfaces. The lower inset: SEM image of the fabric. Scale bar: 200 μm. (E) Photos and thermal images of the flat sample (upper panel) and the “umbrella” structure (lower panel) under one sun illumination. (F) Mass change of the three structures over 8 h using fresh water under one sun illumination. Image Credit: Kunjaram, et al., 2022
Methodology
The tapered evaporation architecture described in the new paper is shown in Figure 1B. Due to the reduced solar energy density on the bigger surface, the surface temperatures of this architecture remain close to or lower than the ambient temperature under ordinary one-sun lighting. As a result, rather than heating the vapor, incoming solar energy was primarily designed to transform liquid water to gas-phase moisture.
Water is delivered from a central vertical channel to the upper surface, as shown in Figure 1C.
As a result, instead of the surface of the evaporative material in the tapered architecture depicted in Figure 1B, water inside the evaporative substance will flow toward the two dead endpoints, evaporate, and deposit salts at the surface of EPE foams.
Researchers created a flat (Figure 1A), a tapered edge to center triangle (Figure 1B), and an “umbrella” evaporation system employing carbon-coated fabric (CCF) as the evaporative interfaces to test this idea.
Results and Discussion
The flat structure and the suggested “umbrella” structures consisting of 1-layer and 12-layers in the two wings were exposed to saltwater to describe the solar-driven evaporation rates. The water’s saltiness was raised from 3.5 wt% to 7 wt% and 10 wt%.
As the salinity rises, the center clean area proportion decreases from 55.2% (Figure 2A) to 35.3% (Figure 2C).
Crystallized salts filled roughly 9% of the surface area in the 7 wt% salt water (as depicted in the second column in Figure 2B), which is significantly less than the salt occupancy rate of 57% on the flat surface (as shown in the first column in Figure 2B). Surprisingly, as seen in the third column of Figure 2B, the salt accumulation problem was eradicated in the 12-layer construction.
Due to no evident salt crystallization, the evaporation rate of the 12-layer umbrella structure is over 2.65 kg m−2 h−1, as represented by red spheres in Figure 2D.
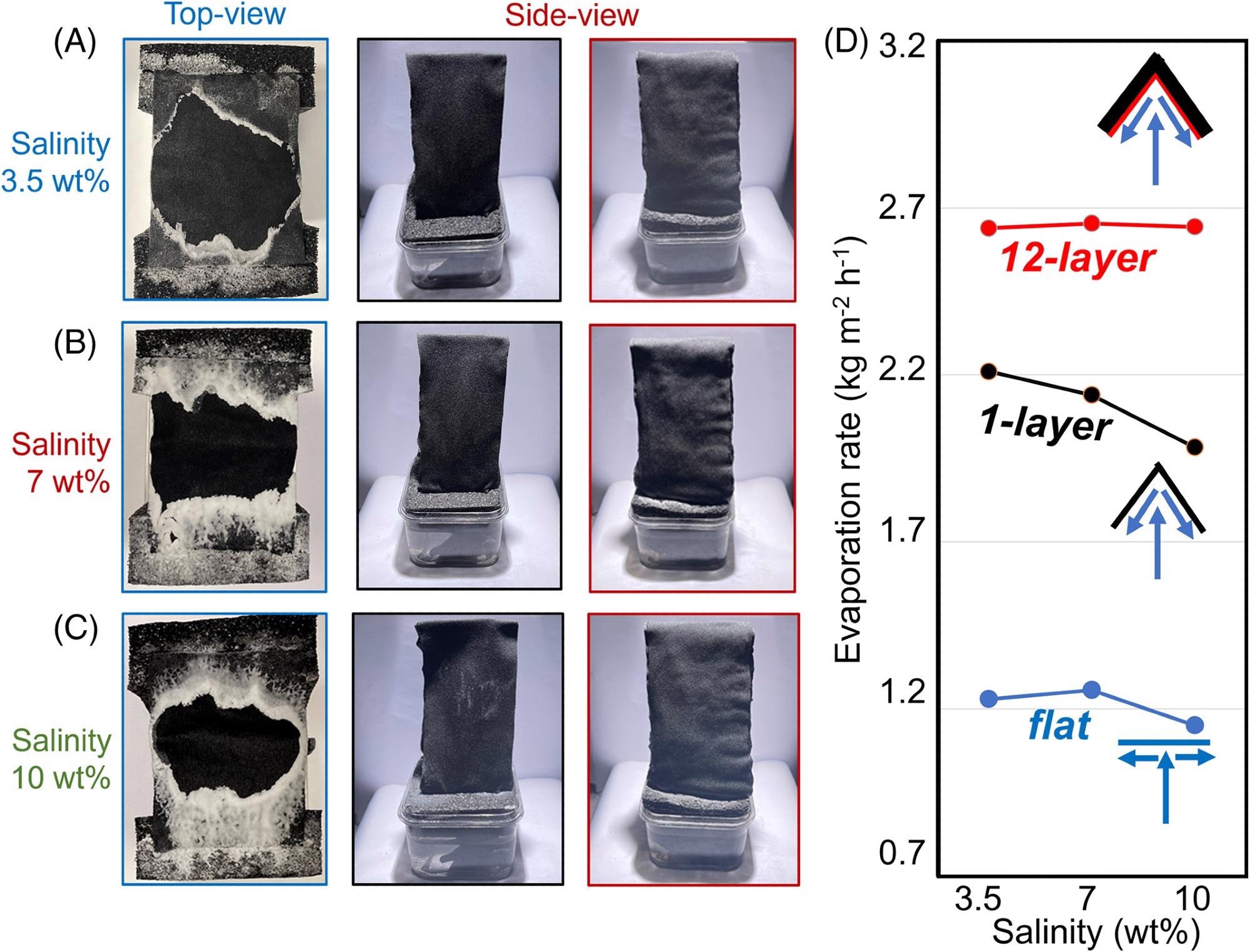
Figure 2. Self-cleaning evaporative architecture. (A–C) Comparison of the three structures (i.e., the first column: Top-view of the flat structure, the second column: side-view of the 1-layer structure, and the third column: side-view of the 12-layer structure) after 10 hours of operation under one sun illumination with the salinity of (A) 3.5 wt%, (B) 7 wt%, and (C) 10 wt%, respectively. (D) Measured evaporation rates under 1 sun illumination. Insets: Architecture of the flat (the lower panel), 1-layer (the central panel), and 12-layer (the upper panel) evaporative structures. Image Credit: Kunjaram, et al., 2022
The water was transferred to the upper surface by the center fabric due to capillary force, as shown in Figure 3A. A large surface evaporation rate, QE, is always desirable for water evaporation purposes. To avoid salt accumulation, the basic goal is to increase water flow rates to reduce local salinity.
The umbrella architecture provided bigger surface areas for improved QE when compared to the flat structure shown in Figure 1A.
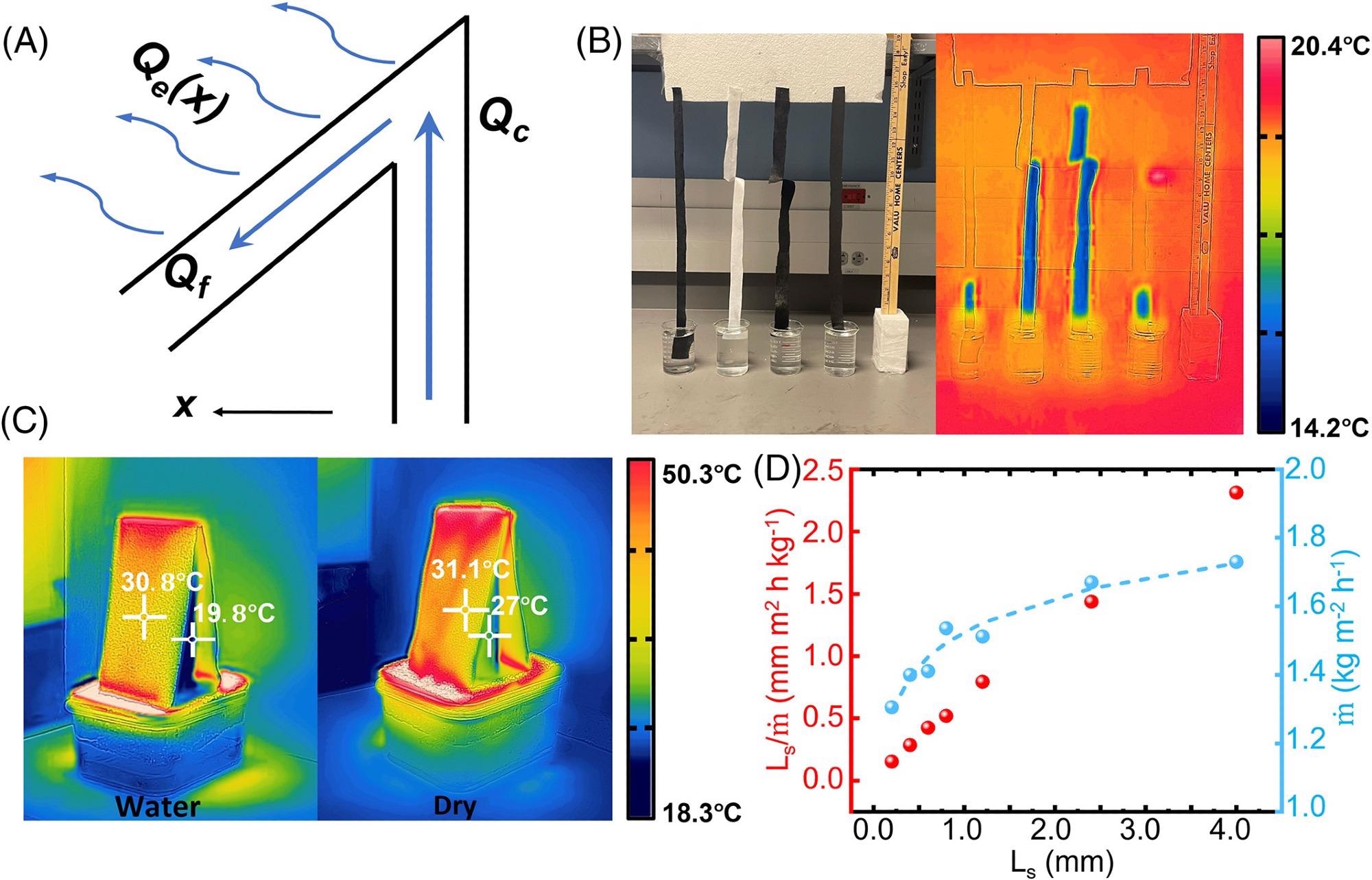
Figure 3. Water transfer capability. (A) Characterization of the salt accumulation on the umbrella structure. (B) Comparison of materials (ProCool Athletic Pique®, Texwipe 609®, CCF, and Highland® fabric) to determine maximum Qc. (C) Thermal images of the 1-layer evaporation surface (the left panel) and the dry surface (the right panel) under the same solar under four sun illumination. The color bar is tuned to better show the temperatures on the evaporators. (D) Measured ratio of Ls/m (red spheres) and m (blue spheres) as the function of the evaporative film thickness Ls. The blue dashed line is the fitting curve to show the trend of Qe with the increasing Ls. Image Credit: Kunjaram, et al., 2022
Researchers chose four samples attached with the lower ends immersed in fresh water and captured their thermal images to examine the water transportation height, as shown in Figure 3B. To adjust the surface temperature and evaporation rate of the umbrella structure, the incoming light strength was raised in Figure 3C.
It should be indicated that due to the Marangoni effect, the temperature difference could possibly produce directed water flow. It has the potential to help prevent salt accumulation, but more research is needed. The local surface evaporation, Qe(x), on the other hand, will lead to a salinity gradient, as seen in Figure 3A.
By adjusting Ls alone for given umbrella constructions in Figure 3D, researchers characterized the entire evaporation rate under one sun illumination.
The photographs in Figure 4A show the construction after a 10-hour operation under one solar illumination for four days. These salt crystals are firmly combined with the carbon powder on the fabric microstructure, as shown in the SEM image of the contaminated surface in Figure 4B, making mechanical removal challenging.
On this structured fabric, bigger salt crystals were seen, as shown in Figure 4C.
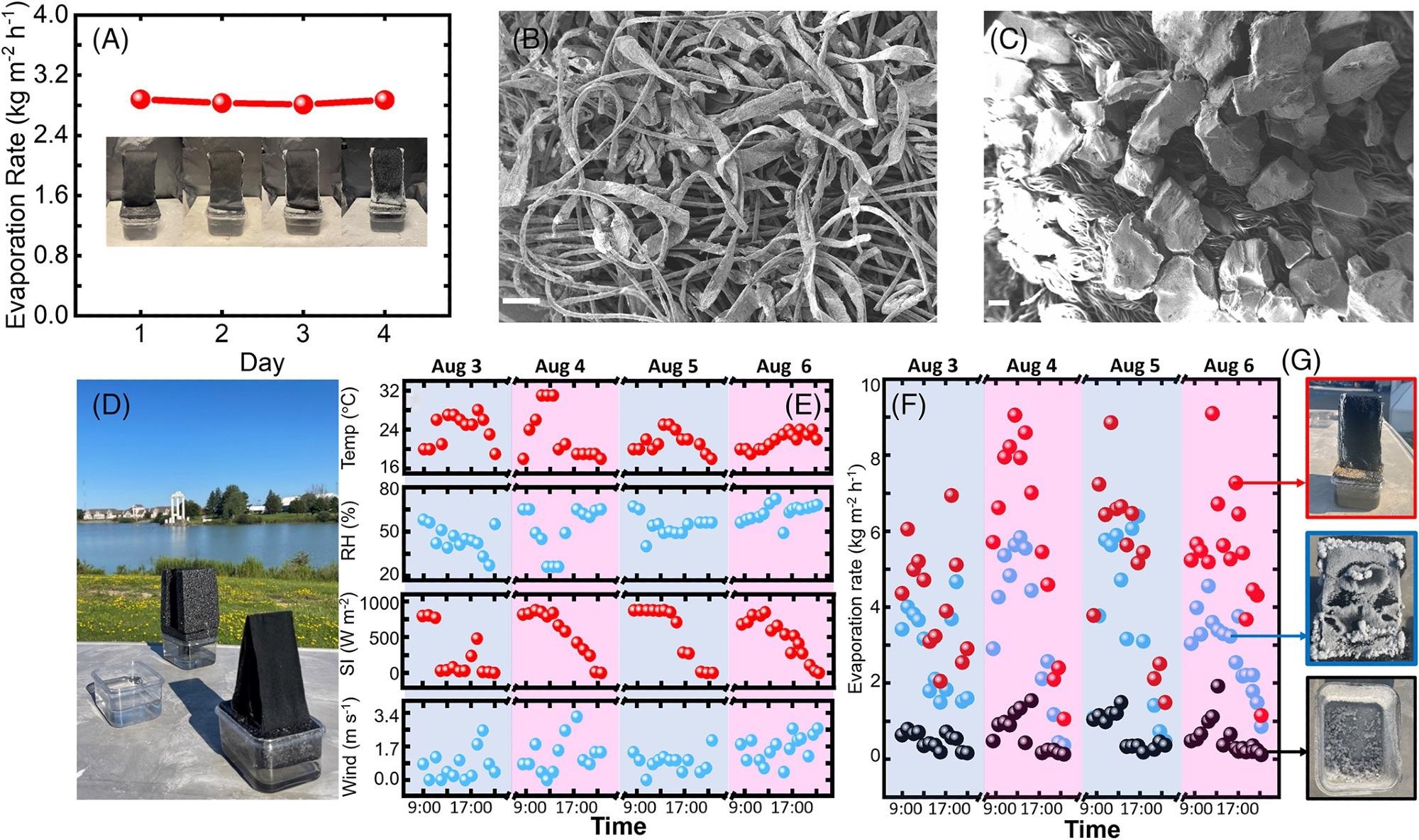
Figure 4. Experimental results for salinity of 20 wt%. (A) Measured evaporation rates of the four-day indoor experiment under one sun illumination. Inset: photos of the umbrella structure on each day. (B, C) SEM images of the accumulated salt on (B) the CCF (scale bar: 100 μm), and (C) the Highland fabric (scale bar: 200 μm), respectively. (D) A photo of the outdoor experiment on August 3rd, 2021, at the University at Buffalo. (E) Measured environmental conditions for the outdoor experiment (from top to bottom): the relative humidity (RH), the temperature, the solar intensity (SI), and the wind velocity. (F) Measured evaporation rates of the umbrella structure (red), the flat structure (blue), and the bulk water surface (black), respectively. (G) Photos of the salt accumulation after the 4-day operation on the umbrella structure (the upper panel), the flat structure (the central panel), and the bottom of the container (the lower panel). Image Credit: Kunjaram, et al., 2022
Lastly, to conduct the field test, researchers placed this umbrella structure, a flat structure, and a beaker containing 20 wt% saltwater in an outdoor setting in Buffalo, NY (Figure 4D).
Figure 4E depicts the weather conditions over four days, including ambient temperature, relative humidity, sun intensity, and wind speed. The evaporation rates of the three components were tested once an hour during the day to capture their weight losses. The greatest evaporation rate of the umbrella structure reaches 9.05 kg m−2 h−1 due to natural wind input, as shown in Figure 4F.
Surprisingly, salts only crystallized along the umbrella structure’s edges, as illustrated in Figure 4G’s upper panel.
This research highlights the ability of the suggested umbrella structures for enhanced salt mining applications formerly provided by solar and wind-driven evaporation procedures.
Conclusion
Finally, researchers described “umbrella” architecture for solar-driven interfacial evaporation that may generate cold vapor while preventing salt accumulation on the evaporative surface. The umbrella architecture has a substantially wider surface area than flat structures, which were frequently used in most solar evaporation studies, resulting in much reduced solar power densities on its wings.
It was discovered that maintaining a suitable amount of water transportability and salt resistance are important elements in reducing local salt saturation.
Surprisingly, most salts crystallized outside of the evaporative surfaces, making salt collection simpler. This type of low-cost “umbrella” architecture appears to have the potential to lay the groundwork for ZLD desalination and faster salt mining technologies.
Journal Reference:
Kunjaram, U. P. U., Song, H., Liu, Y., Booker, B. K., Cooke, T. J., Gan, Q. (2022) A self‐salt‐cleaning architecture in cold vapor generation system for hypersaline brines. EcoMat, 4(2), p. e12168. Available Online: https://onlinelibrary.wiley.com/doi/10.1002/eom2.12168.
References and Further Reading
- Tao P., et al. (2018) Solar-driven interfacial evaporation. Nature Energy, 3, pp. 1031–1041. doi.org/10.1038/s41560-018-0260-7.
- Elimelech M & Phillip W A (2011) The future of seawater desalination: energy, technology, and the environment. Science, 333(6043), pp. 712–717. doi.org/10.1126/science.1200488.
- Shi J., et al. (2021) Efficient and antifouling interfacial solar desalination guided by a transient salt capacitance model. Cell Reports, 2(2), p. 100330. .doi.org/10.1016/j.xcrp.2021.100330.
- Zhang Y., et al. (2020) Structure architecting for salt-rejecting solar interfacial desalination to achieve high-performance evaporation with in situ energy generation. Advanced Science, 7, p. 1903478. doi.org/10.1002/advs.201903478.
- Fang Q., et al. (2019) Highly efficient solar steam generation from activated carbon fiber cloth with matching water supply and durable fouling resistance. ACS Applied Energy Materials, 2(6), pp. 4354– 4361. doi.org/10.1021/acsaem.9b00562.
- Jin Y., et al. (2018) A highly flexible and washable nonwoven photothermal cloth for efficient and practical solar steam generation. Journal of Materials Chemistry, 6(17), pp. 7942–7949. doi.org/10.1039/C8TA00187A.
- Zhang C., et al. (2021) Designing a next generation solar crystallizer for real seawater brine treatment with zero liquid discharge. Nature Communications, 12, p. 998. doi.org/10.1038/s41467-021-21124-4.
- Wang Y., et al. (2018) Improved light-harvesting and thermal management for efficient solar-driven water evaporation using 3D photothermal cones. Journal of Materials Chemistry, 6(21), pp. 9874–9881. doi.org/10.1039/C8TA01469H.
- Wu X., et al. (2021) Dual-zone photothermal evaporator for antisalt accumulation and highly efficient solar steam generation. Advanced Functional Materials, 31, p. 2102618. doi.org/10.1002/adfm.202102618.
- Xia Y., et al. (2019) Spatially isolating salt crystallisation from water evaporation for continuous solar steam generation and salt harvesting. Energy & Environmental Science,12(6), pp. 1840–1847. doi.org/10.1039/C9EE00692C.
- Zang L, et al. (2021) Interfacial solar vapor generation for desalination and brine treatment: evaluating current strategies of solving scaling. Water Research, 198, p. 117135. doi.org/10.1016/j.watres.2021.117135.
- Fan Y., et al. (2021) Enhanced solar-to-heat efficiency of photothermal materials containing an additional light-reflection layer for solar-driven interfacial water evaporation. ACS Applied Energy Materials, 4(3), pp. 2932–2943. doi.org/10.1021/acsaem.1c00391.
- He S., et al. (2019) Nature-inspired salt resistant bimodal porous solar evaporator for efficient and stable water desalination. Energy & Environmental Science, 12(5), pp. 1558–1567. doi.org/10.1039/C9EE00945K.
- Hu C.-S., et al. (2019) Mushroom-like rGO/PAM hybrid cryogels with efficient solar-heating water evaporation. ACS Applied Energy Materials, 2(10), pp. 7554–7563. doi.org/10.1021/acsaem.9b01530.
- Kuang Y., et al. (2019) A high-performance self-regenerating solar evaporator for continuous water desalination. Journal of Science: Advanced Materials,31, p. 1900498. https://onlinelibrary.wiley.com/journal/15214095.
- Xu K, et al. (2021) Salt mitigation strategies of solar-driven interfacial desalination. Advanced Functional Materials, 31, p. 2007855. doi.org/10.1002/adfm.202007855.
- Wang W., et al. (2021) Integrated solar-driven PV cooling and seawater desalination with zero liquid discharge. Joule,5(7), pp. 1873–1887. doi.org/10.1016/j.joule.2021.05.010.
- Xia Y., et al. (2021) Rational designs of interfacial-heating solar-thermal desalination devices: recent progress and remaining challenges. Journal of Materials Chemistry,9(11), pp. 6612–6633. doi.org/10.1039/D0TA11911C.
- Zhang L., et al. (2021) Passive, high-efficiency thermally-localized solar desalination. Energy & Environmental Science,14(4), pp. 1771–1793. doi.org/10.1039/D0EE03991H.
- Ghasemi H., et al. (2014) Solar steam generation by heat localization. Nature Communications, 5, pp. 1–7. doi.org/10.1038/ncomms5449.
- Zhou L., et al. (2021) Hybrid concentrated radiative cooling and solar heating in a single system. Cell Reports, 2(2), p. 100338. doi.org/10.1016/j.xcrp.2021.100338.
- Zhou L., et al., (2019) Apolydimethylsiloxane-coated metal structure for all-day radiative cooling. Nature Sustainability, 2, pp. 718– 724. doi.org/10.1038/s41893-019-0348-5.
- Zhou M., et al. (2021) Vapor condensation with daytime radiative cooling. Proceedings of the National Academy of Sciences of the United States of America, 118(14). doi.org/10.1073/pnas.2019292118.
- Wu L., et al. (2020) Highly efficient three-dimensional solar evaporator for high salinity desalination by localized crystallization. Nature Communications, 11, p. 521. doi.org/10.1038/s41467-020-14366-1.
- Liu Z., et al. (2017) Extremely cost-effective and efficient solar vapor generation under nonconcentrated illumination using thermally isolated black paper. Global Challenges,1, p. 1600003. https://onlinelibrary.wiley.com/journal/20566646.
- Ni G., et al. (2018) A salt-rejecting floating solar still for low-cost desalination. Energy & Environmental Science, 11(6), pp. 1510–1519. doi.org/10.1039/C8EE00220G.
- Zhu L., et al. (2019) Shape conformal and thermal insulative organic solar absorber sponge for photothermal water evaporation and thermoelectric power generation. Advanced Energy Materials, 9, p. 1900250. doi.org/10.1002/aenm.201900250.
- Zou M., et al. (2021) 3D printing a biomimetic bridge-arch solar evaporator for eliminating salt accumulation with desalination and agricultural applications. Advanced Materials, 33(34), p. e2102443. doi.org/10.1002/adma.202102443.
- Lu Y.,et al. (2021) Coupling solar-driven photothermal effect into photocatalysis for sustainable water treatment. Journal of Hazardous Materials,423, p. 127128. doi.org/10.1016/j.jhazmat.2021.127128.
- Fan D., et al. (2021) Synergy of photocatalysis and photothermal effect in integrated 0D perovskite oxide/2D MXeneheterostructures for simultaneous water purification and solar steam generation. Applied Catalysis, 295, p. 120285. doi.org/10.1016/j.apcatb.2021.120285.
- Li O L., et al. (2019) Effect of hydrophilic/hydrophobic properties of carbon materials on plasma-sulfonation process and their catalytic activities in cellulose conversion. Catalysis Today, 337, pp. 155–161. doi.org/10.1016/j.cattod.2019.04.025.
- Wang X., et al. (2020) Water delivery channel design in solar evaporator for efficient and durable water evaporation with salt rejection. ACS Sustainable Chemistry & Engineering; 8(21), pp. 7753–7761. doi.org/10.1021/acssuschemeng.9b06844.
- Liu H., et al. (2021) Anisotropic evaporator with a T-shape design for high-performance solar-driven zero-liquid discharge. Small,17, p. e2100969. doi.org/10.1002/smll.202100969.
- Chen C., et al. (2017) Highly flexible and efficient solar steam generation device. Journal of Science: Advanced Materials, 29, p. 1701756. doi.org/10.1002/adma.201701756.
- Zhu M., et al. (2018) Plasmonic wood for high-efficiency solar steam generation. Advanced Energy Materials,8, p. 1701028. doi.org/10.1002/aenm.201701028.
- Zhu M., et al. (2017) Tree-inspired design for high-efficiency water extraction. Journal of Science: Advanced Materials,29, p. 1704107. doi.org/10.1002/adma.201704107.
- Shi Y., et al. (2018) Solar evaporator with controlled salt precipitation for zero liquid discharge desalination. Environmental Science & Technology,52(20), pp. 11822–11830. doi.org/10.1021/acs.est.8b03300.
- Wang W., et al. (2021) Solar seawater distillation by flexible and fully passive multistage membrane distillation. Nano Letters,21(12), pp. 5068–5074. doi.org/10.1021/acs.nanolett.1c00910.
- Yang L., et al. (2021) Photovoltaic-multistage desalination of hypersaline waters for simultaneous electricity, water and salt harvesting via automatic rinsing. Nano Energy, 87, p. 106163. doi.org/10.1016/j.nanoen.2021.106163.
- Li X., et al. (2018) Three-dimensional artificial transpiration for efficient solar waste-water treatment. National Science Review, 5(1), pp. 70–77. doi.org/10.1093/nsr/nwx051.
- Xu N., et al. (2021) A scalable fish-school inspired self-assembled particle system for solar-powered water-solute separation. National Science Review, 8(10), p. nwab065. doi.org/10.1093/nsr/nwab065.
- Lu Y., et al. (2020) Implementing hybrid energy harvesting in 3D spherical evaporator for solar steam generation and synergic water purification. Solar RRL,4, p. 2000232. https://doi.org/10.1002/solr.202000232.
- Song H., et al. (2018) Cold vapor generation beyond the input solar energy limit. Advanced Science, 5, p. 1800222. doi.org/10.1002/advs.201800222.
- Zhao F., et al. (2018) Highly efficient solar vapour generation via hierarchically nanostructured gels. Nature Nanotechnology,13(6), pp. 489–495. doi.org/10.1038/s41565-018-0097-z.
- Guo Y., et al. (2020) Biomass-derived hybrid hydrogel evaporators for cost-effective solar water purification. Journal of Science: Advanced Materials, 32, p. 1907061.doi.org/10.1002/adma.201907061.
- Zhou X., et al. (2018) A hydrogel-based antifouling solar evaporator for highly efficient water desalination. Energy & Environmental Science,11(8), pp. 1985–1992. doi.org/10.1039/C8EE00567B.
- Lu Y., et al. (2021) Surface patterning of two-dimensional nanostructure-embedded photothermal hydrogels for high-yield solar steam generation. ACS Nano, 15(6), pp. 10366–10376. doi.org/10.1021/acsnano.1c02578.
- Highland, (2021) https://highlandindustries.com/products/.
- Werber JR., et al. (2016) Materials for next-generation desalination and water purification membranes. Nature Reviews Materials, 1, pp. 1–15. doi.org/10.1038/natrevmats.2016.18.
- Brika B (2016) Environmental implications of Tajoura reverse osmosis desalination plant. Desalination and Water Treatment, 57(46), pp. 21712–21720. doi.org/10.1080/19443994.2015.1130920.
- Akinaga T., et al. (2018) Brine utilisation for cooling and salt production in wind-driven seawater greenhouses: design and modelling. Desalin, 426, pp. 135–154. doi.org/10.1016/j.desal.2017.10.025.
- Loganathan P., et al. (2017) Mining valuable minerals from seawater: a critical review. Environmental Science: Water Research & Technology, 3(1), pp. 37–53. doi.org/10.1039/C6EW00268D.