Human activities have caused a spike in greenhouse gas concentrations since pre-industrial times, which is the primary cause of global warming. Different climatic intervention methods have been proposed to limit global warming. Solar radiation modification (SRM, also known as geoengineering) is one of them. It aims to cool the Earth by intentionally reducing shortwave radiation reaching the surface to neutralize some of the warming effects of greenhouse emissions.
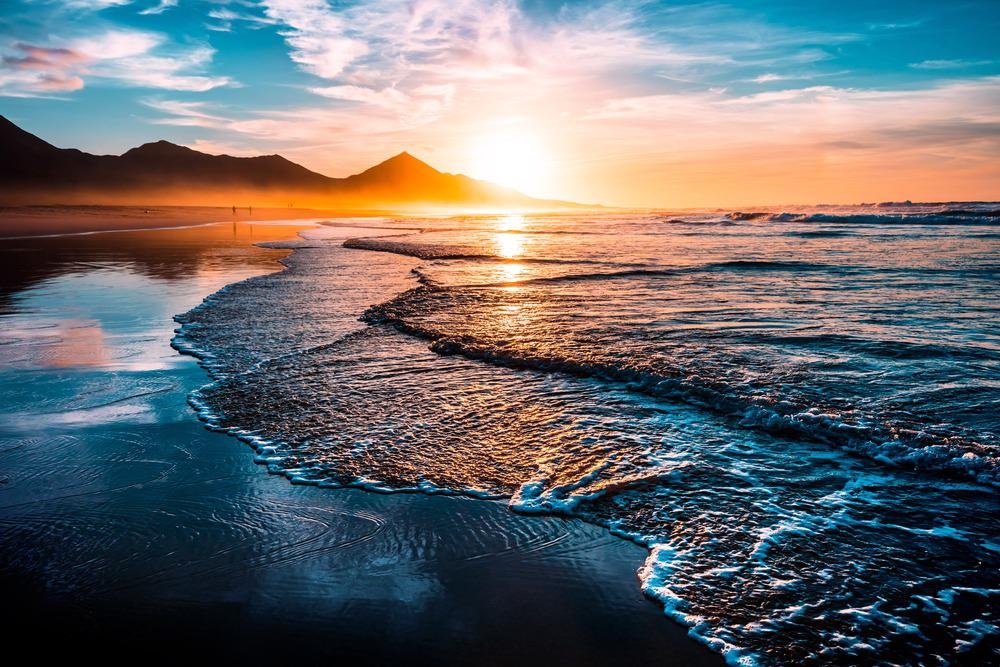
Image Credit: Don Pablo/Shutterstock.com
Most existing studies have concentrated on the impact of SRM on the physical properties of the climate system such as precipitation, temperature, ocean circulation, and sea ice. However, the impact of SRM on the carbon cycle has received far less attention.
Researchers have built on past studies to explore the impact of SRM on the ocean carbon cycle and marine biogeochemistry. They used an Earth system model to run idealized SRM simulations in which solar irradiance is evenly lowered to decrease the global mean temperature from a high-CO2 emission situation to a low-CO2 emission scenario. The paper was published in Atmospheric and Oceanic Science Letters.
The study looked at the impact of reduced solar radiation on a variety of ocean carbon cycle variables ranging from seasonal to centennial time scales, as well as the fundamental governing procedures. The research advances our insight into the potential effects of SRM on ocean biogeochemical cycles and the marine environment.
Methodology
The University of Victoria Earth System Climate Model (ESCM) version 2.10 was used in this research, which is an Earth system model of transitional complexity that pairs the global climate and the carbon cycle. A land surface and terrestrial carbon cycle model, a three-dimensional ocean general circulation model, and a vertically integrated energy–moisture balance atmospheric model comprise the model.
The model ran for 10,000 years at a pre-industrial CO2 concentration of 280 ppm. After a 10,000-year simulation, the model attained a quasi-equilibrium state for the global climate and the carbon cycle.
Results and Discussion
As shown in Figure 1a, a uniform reduction in solar insolation under RCP8.5 (RCP8.5_SRM) reduces the global mean surface air temperature to that of RCP4.5.
However, there is a notable change in the spatial pattern of surface temperature between RCP4.5 and RCP8.5_SRM.
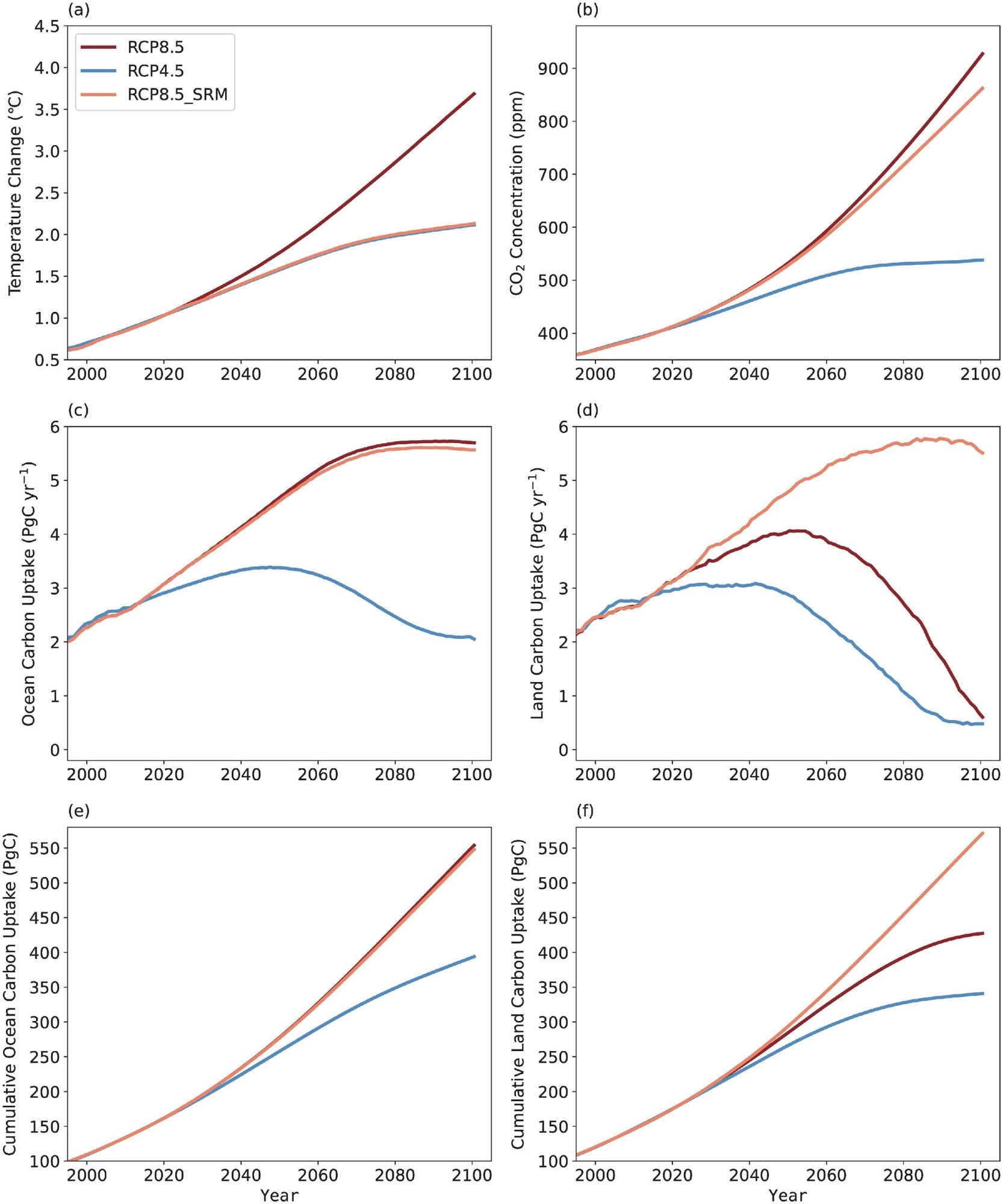
Figure 1. Model-simulated time series of temperature and carbon cycle variables for the scenarios of RCP4.5, RCP8.5, and RCP8.5_SRM: (a) change in global mean surface air temperature (relative to 1850); (b) atmospheric CO2 concentration; (c) annual CO2 uptake by the ocean; (d) annual CO2 uptake by the land; (e) cumulative CO2 uptake by the ocean; (f) cumulative CO2 uptake by the land. Image Credit: Jin, et al. 2022
Figure 1b shows the simulated atmospheric CO2 levels in the year 2100.
In Figure 2a, the total amount of anthropogenic carbon (in this, the term anthropogenic carbon refers to the variation in carbon storage (or flux) relative to the preindustrial state) contained in the terrestrial ecosystem by the year 2100 is 341, 427, and 571 PgC for RCP4.5, RCP8.5, and RCP8.5_SRM, respectively.
SRM-induced cooling also reduces soil respiration. As a result, in Figure 1d, the net terrestrial CO2 uptake, which is the balance between NPP and soil respiration, is higher in RCP8.5_SRM than in RCP8.5, increasing net terrestrial carbon storage in huge portions of land regions.
Moreover, as shown in Figure 1b, the increased CO2 absorption by the terrestrial biosphere in RCP8.5_SRM results in a reduction in atmospheric CO2.
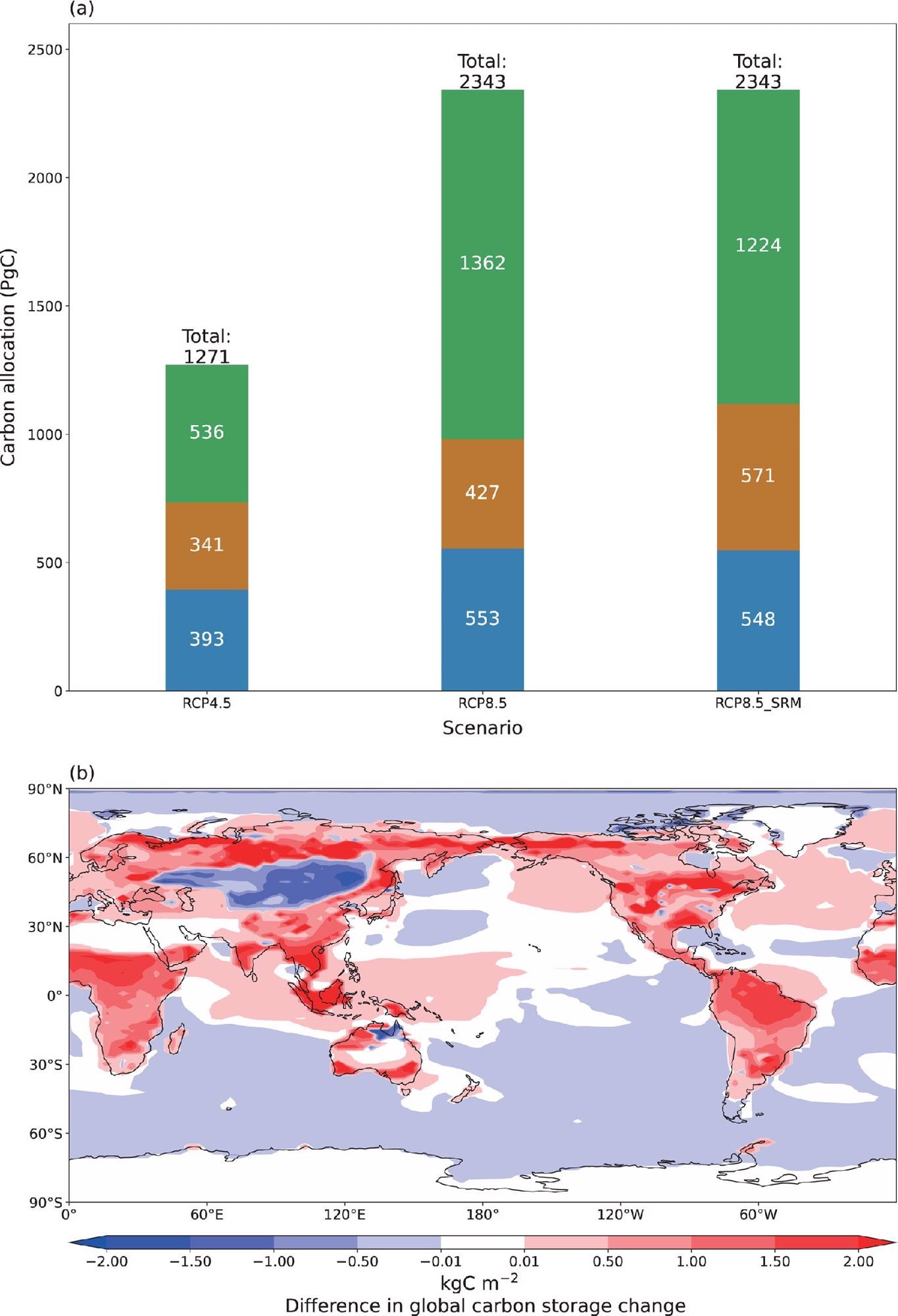
Figure 2. (a) Model-simulated allocation of anthropogenic carbon emissions for the scenarios of RCP4.5, RCP8.5, and RCP8.5_SRM for the year 2100. (b) Spatial pattern of the difference in global carbon storage for RCP8.5_SRM relative to RCP8.5. Values were calculated using the simulation results averaged over 2090–2100. Image Credit: Jin, et al. 2022
Figure 2a depicts the total amount of anthropogenic carbon stored by the ocean by the year 2100.
Ultimately, the impact of reduced atmospheric CO2 and SRM-induced cooling act with each other in terms of oceanic CO2 uptake, leading to a small change in global ocean carbon storage in Figure 2b.
Figure 2a depicts the allocation of anthropogenic CO2 in various scenarios.
CO2-induced ocean acidification is predicted to have a wide range of negative consequences for the marine ecosystem. The global ocean becomes more acidic as a result of anthropogenic CO2 uptake.
In Figure 3a, the model simulated the yearly and global mean surface hydrogen ion concentration [H+] enhances by 67%, 168%, and 151% relative to the pre-industrial state for RCP4.5, RCP8.5, and RCP8.5_SRM, respectively.
![Model-simulated temporal evolution of key carbonate chemistry variables for the scenarios of RCP4.5, RCP8.5, and RCP8.5_SRM: (a–c) the global mean surface ocean (a) [H+], (b) pH, and (c) aragonite saturation, in which annual mean values are overplotted with monthly mean values; (d–f) the global mean change in the seasonal amplitude of (d) [H+], (e) pH, and (f) aragonite saturation.](https://d3hnfqimznafg0.cloudfront.net/images/Article_Images/ImageForArticle_1461_16461170151092755.jpg)
Figure 3. Model-simulated temporal evolution of key carbonate chemistry variables for the scenarios of RCP4.5, RCP8.5, and RCP8.5_SRM: (a–c) the global mean surface ocean (a) [H+], (b) pH, and (c) aragonite saturation, in which annual mean values are overplotted with monthly mean values; (d–f) the global mean change in the seasonal amplitude of (d) [H+], (e) pH, and (f) aragonite saturation. Image Credit: Jin, et al. 2022
Figure 3d–f depicts the researchers’ assessment of the simulated variations in the seasonality of the ocean carbonate chemistry variables.
In Figure 3f, the mean seasonal amplitude of the aragonite saturation state is lowered by 16%, 30%, and 31% by the year 2100 for RCP4.5, RCP8.5, and RCP8.5 SRM, respectively.
NPP is an incorporated indicator of ocean ecosystem productivity and a key component of the biological pump that regulates CO2 uptake in the ocean. NPP reduces by 1.8% under RCP8.5 SRM, similar to RCP4.5 in Figure 4a. These findings point to a high level of uncertainty in model-simulated ocean NPP change.
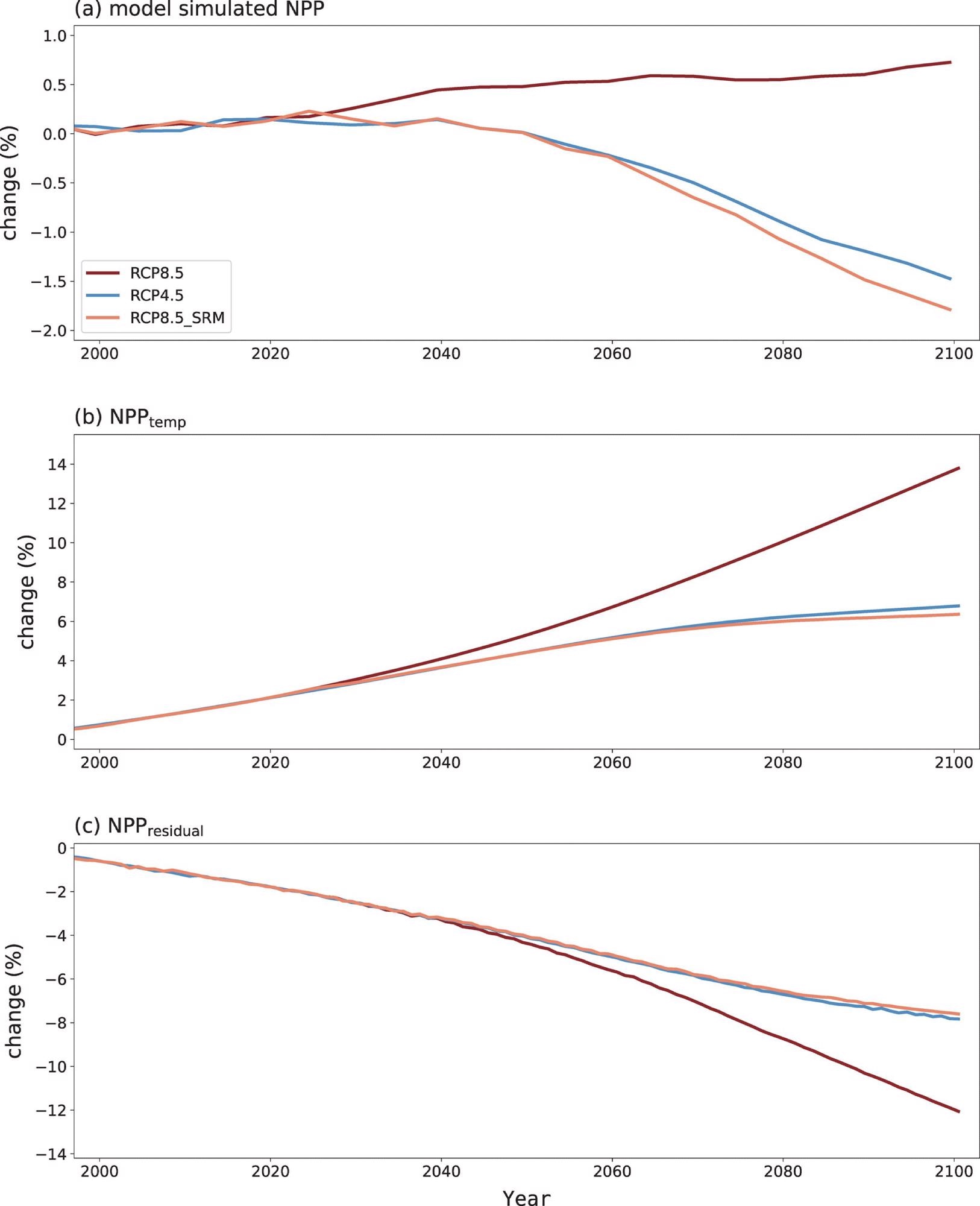
Figure 4. Time series of annual and global mean ocean NPP for the scenarios of RCP4.5, RCP8.5, and RCP8.5_SRM. All results are plotted as the percentage change relative to the year 1850. As a reference, the model-simulated ocean NPP in the year 1850 is 67.8 PgC yr−1. (a) NPP from model-simulation results. (b, c) NPP change due to individual factors diagnosed from offline calculations. NPPtemp represents the NPP change due to temperature changes only, and NPPresidual (NPPtotal − NPPtemp − NPPlight) represents the NPP change due to changes in circulation-induced nutrient change. Image Credit: Jin, et al. 2022
Offline simulations show that increasing upper-ocean temperature results in a significant increase in ocean NPP due to the warming-induced increase in the phytoplankton growth rate. Limited nutrient concentrations in the upper ocean correlated with greater ocean stratification, but, on the other hand, it reduced ocean NPP by 12.4% (Figure 4b, c).
Conclusion
The UVic model was used in this research to determine the reaction of the ocean carbon cycle and acidification to SRM. The SRM approach is intended in a highly idealized position to minimize global mean temperature from RCP8.5 to RCP4.5 by evenly reducing solar insolation around the world.
Researchers investigated the impact of rising CO2 and SRM on seasonal changes in ocean acidification. It was discovered that rising atmospheric CO2 levels are the primary cause of changes in the seasonality of ocean acidification.
CO2-induced warming also has an impact on ocean NPP, primarily through increased SST, which enhances phytoplankton growth rates and indirectly through modifications in upper-ocean nutrient concentrations connected with ocean stratification. Moreover, change in NPP is more heterogeneous at regional scales, implying varying abilities of the effects of temperature and ocean mixing on NPP in different areas.
More research using multiple models is required to reduce the unpredictability of the consequences of SRM on the ocean carbon cycle and biogeochemistry.
Finally, the findings highlight the potential effects of SRM on the ocean carbon cycle, ocean acidification, and marine NPP, assisting in the establishment of a robust assessment of SRM’s climatic and environmental impacts.
Journal Reference
Jin, X., Cao, L., Zhang, J. (2022) Effects of solar radiation modification on the ocean carbon cycle: An earth system modeling study. Atmospheric and Oceanic Science Letters, 100187. Available Online: https://www.sciencedirect.com/science/article/pii/S1674283422000526.
References and Further Reading
- Bindoff, N. L., et al. (2019) Changing ocean, marine ecosystems, and dependent communities. IPCC Special Report on the Ocean and Cryosphere in a Changing Climate, In press, pp. 447–587.
- Bopp, L., et al. (2013) Multiple stressors of ocean ecosystems in the 21st century: projections with CMIP5 models. Biogeosciences, 10, pp. 6225–6245. doi.org/10.5194/bg-10-6225-2013.
- Cao, L (2018) The effects of solar radiation management on the carbon cycle. Current Climate Change Reports, 4, pp. 41–50. doi.org/10.1007/s40641-018-0088-z.
- Cao, L., et al. (2007) Effects of carbon dioxide and climate change on ocean acidification and carbonate mineral saturation. Geophysical Research Letters, 34, p. L05607, doi.org/10.1029/2006GL028605.
- Cao, L & Jiang, J (2017) Simulated effect of carbon cycle feedback on climate response to solar geoengineering. Geophysical Research Letters, 44, pp. 12484–12491, doi.org/10.1002/2017GL076546.
- Crutzen, P J (2006) Albedo enhancement by stratospheric sulfur injections: a contribution to resolve a policy dilemma? Climatic Change, 77, p. 211. doi.org/10.1007/s10584-006-9101-y.
- Duan, L., et al. (2020) A model-based investigation of terrestrial plant carbon uptake response to four radiation modification approaches. Journal of Geophysical Research: Atmospheres, 125, p. e2019JD031883. doi.org/10.1029/2019JD031883.
- Duan, L., et al. (2018) Comparison of the fast and slow climate response to three radiation management geoengineering schemes. Journal of Geophysical Research: Atmospheres, 123, pp. 11980–12001. doi.org/10.1029/2018JD029034.
- Egleston, E. S., et al. (2010) Revelle revisited: Buffer factors that quantify the response of ocean chemistry to changes in DIC and alkalinity. Global Biogeochemical Cycles, 24, p. GB1002. doi.org/10.1029/2008GB003407.
- Glienke, S., et al. (2015) The impact of geoengineering on vegetation in experiment G1 of the GeoMIP. Journal of Geophysical Research: Atmospheres, 120, pp. 10196–10213. doi.org/10.1002/2015JD024202.
- Hauck, J & Voelker, C (2015) Rising atmospheric CO2 leads to large impact of biology on Southern Ocean CO2 uptake via changes of the Revelle factor. Geophysical Research Letters, 42, pp. 1459–1464. doi.org/10.1002/2015GL063070.
- IPCC (2021) IPCC (Intergovernmental Panel on Climate Change) Climate Change 2021: The Physical Science Basis. Contribution of Working Group I to the Sixth Assessment Report of the Intergovernmental Panel on Climate Change. Cambridge University Press, London.
- Irvine, P. J., et al. (2016) An overview of the Earth system science of solar geoengineering. WIREs. Climatic Change, 7, pp. 815–833.
- Kalidindi, S., et al. (2015) Modeling of solar radiation management: a comparison of simulations using reduced solar constant and stratospheric sulphate aerosols. Climate Dynamics, 44, pp. 2909–2925. doi.org/10.1007/s00382-014-2240-3.
- Kravitz, B., et al. (2013) Climate model response from the Geoengineering Model Intercomparison Project (GeoMIP). Journal of Geophysical Research: Atmospheres,118, pp. 8320–8332. doi.org/10.1002/jgrd.50646.
- Kwiatkowski, L & Orr, J C (2018) Diverging seasonal extremes for ocean acidification during the twenty-first century. Nature Climate Change, 8, pp. 141–145. doi.org/10.1038/s41558-017-0054-0.
- Kwiatkowski, L., et al (2020) Twenty-first century ocean warming, acidification, deoxygenation, and upper-ocean nutrient and primary production decline from CMIP6 model projections. Biogeosciences, 17, pp. 3439–3470. doi.org/10.5194/bg-17-3439-2020.
- Lauvset, S. K., et al. (2017) Climate engineering and the ocean: effects on biogeochemistry and primary production. Biogeosciences, 14, pp. 5675–5691, doi.org/10.5194/bg-14-5675-2017.
- Lawrence, M. G., et al. (2018) Evaluating climate geoengineering proposals in the context of the Paris Agreement temperature goals. Nature Communications, 9, p. 3734, doi.org/10.1038/s41467-018-05938-3.
- Mengis, N., et al. (2020) Evaluation of the University of Victoria Earth System Climate Model version 2.10 (UVic ESCM 2.10) Geoscientific Model Development, 13, pp. 4183-4204. doi.org/10.5194/gmd-13-4183-2020.
- Muri, H., et al. (2018) Climate response to aerosol geoengineering: a multimethod comparison. Journal of Climate, 31, pp. 6319–6340. doi.org/10.1175/JCLI-D-17-0620.1.
- Niemeier, U & Timmreck, C (2015) What is the limit of climate engineering by stratospheric injection of SO2? Atmospheric Chemistry and Physics, 15, pp. 9129–9141. doi.org/10.5194/acp-15-9129-2015.
- Park, C. E., et al. (2019) Inequal responses of drylands to radiative forcing geoengineering methods. Geophysical Research Letters, 46, pp. 14011–14020, doi.org/10.1029/2019GL084210.
- Stjern, C. W., et al. (2018) Response to marine cloud brightening in a multi-model ensemble. Atmospheric Chemistry and Physics, 18, pp. 621–634. doi.org/10.5194/acp-18-621-2018.
- Thornton, P. E., et al. (2009) Carbon-nitrogen interactions regulate climate-carbon cycle feedbacks: results from an atmosphere-ocean general circulation model. Biogeosciences, 6, pp. 2099–2120. doi.org/10.5194/bg-6-2099-2009.
- Tjiputra, J. F., et al (2010) Anthropogenic carbon dynamics in the changing ocean. Ocean Sciences, 6, pp. 605–614. doi.org/10.5194/os-6-605-2010.
- Tjiputra, J. F., et al. (2016) Impact of idealized future stratospheric aerosol injection on the large-scale ocean and land carbon cycles. Journal of Geophysical Research: Biogeosciences, 121, pp. 2–27. doi.org/10.1002/2015jg003045.
- Wang, Z. A., et al. (2017) Seasonal controls of aragonite saturation states in the Gulf of Maine. Journal of Geophysical Research: Oceans, 122, pp. 372–389. doi.org/10.1002/2016JC012373.
- Weaver, A. J., et al. (2001) The UVic Earth System Climate Model: Model description, climatology, and applications to past, present and future climates. Atmosphere-Ocean, 39, pp. 361–428. doi.org/10.1080/07055900.2001.9649686.
- Westberry, T., et al. (2008) Carbon-based primary productivity modeling with vertically resolved photoacclimation. Global Biogeochemical Cycles, 22, p. GB2024. doi.org/10.1029/2007GB003078.
- Yang, C. E., et al. (2020) Assessing terrestrial biogeochemical feedbacks in a strategically geoengineered climate. Environmental Research Letters, 15, p. 104043, doi.org/10.1088/1748-9326/abacf7