Climate change is now generally accepted as humanity’s most serious contemporary challenge. According to a report released by the Intergovernmental Panel on Climate Change (IPCC), the situation has worsened, with 3.3 billion people globally vulnerable to climate change. This article looks at microbial responses to climate change in three major ecosystems, with a summary of research published in mBio.
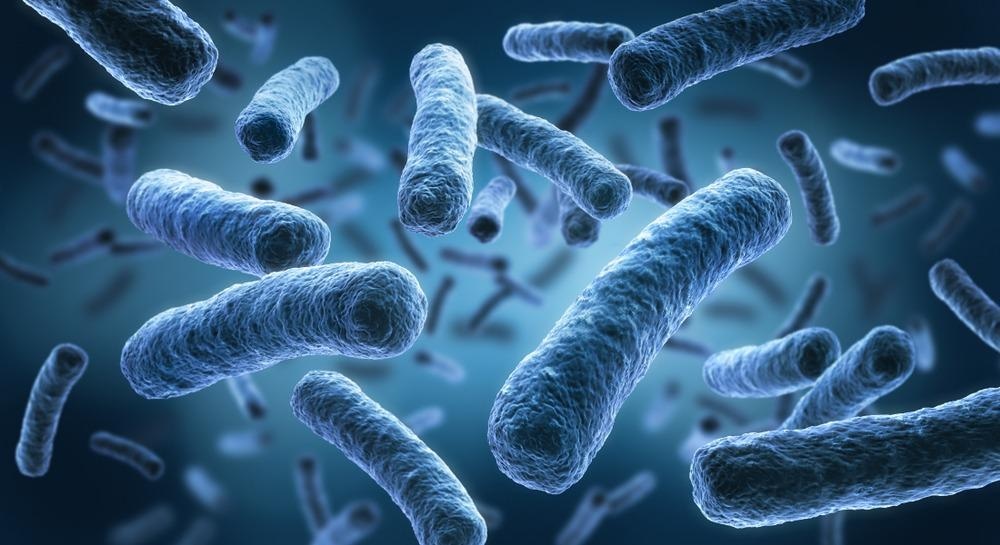
Image Credit: peterschreiber.media/Shutterstock.com
Microbes play an essential role in climate change. They consume and produce the three dominant gases—methane (CH4), carbon dioxide (CO2), and nitrous oxide (N2O)—responsible for 98% of the warming.
Microbes that produce and consume these gases live in various environments, each with its own spatial scales and processing times, making identification difficult. Terrestrial, oceanic, and urban ecosystems present individual assessment and management challenges. Improved measurements and models are essential for determining greenhouse gas (GHG) fluxes in these various systems.
Apart from their direct role with GHG, microbes with pathogenic ability respond to climate change by extending their ranges.
On November 5th, 2021, the American Academy of Microbiology held a colloquium to discuss the changing relationship between microbes, climate change, and cascading effects. The authors were present at the colloquium. The current study expands on some of the concepts discussed at the colloquium, offering an expanded perspective and viewpoints on some of the needed research to fill knowledge gaps.
Discussion
Soil microbes play crucial roles in controlling Earth’s climate by regulating the turnover of soil organic matter (SOM), the biggest organic carbon pool in the terrestrial biosphere. The effects of climate change on soil microbes are expected to vary significantly across ecosystems.
Information on microbes’ temporal dynamics in response to climate change is rarely available. Various soil microbes (archaea, bacteria, fungi, viruses, and protists) and microfauna in both subsoil and topsoil would be able to respond to myriad climate change factors in microbe-centric experiments (elevated CO2, warming, increased precipitation, nutrient addition, drought, and their interactions).
Microbe-centric experiments should be combined with existing infrastructures such as the National Ecological Observatory Network (NEON), university research stations, and Long-Term Ecological Research (LTER) to lower experimental costs. In addition, a global consortium should be formed to coordinate research efforts by ensuring that experimental treatments, sampling protocols, and measurements are all consistent or identical.
The ocean covers 70% of the planet and reaches a depth of 4000 m. Oceans play an essential role in global climate change. Since the industrial revolution, they have absorbed over 90% of the heat accumulating in the atmosphere and 25% of the excess carbon dioxide, leading to ocean acidification.
Since the ecological consequences of ocean acidification and warming are largely irreversible, scientists must develop a clear understanding of microbes and climate change. To accurately predict the effects of climate change, new biogeochemical models will need to consider the resiliency of varied microbial communities and the interactions of multiple drivers. Detailed research into the microbial ecology of marine heatwaves would be a great addition to this future research prospectus.
The ascent of megacities with significant carbon footprints is fueling feedback loops that amplify climate change’s adverse effects, such as disease outbreaks. An appealing economic response is converting greenhouse gases into feedstocks inside a circular economy, where resources retrieved from waste become feedstocks for renewable energy and essential products.
Aboveground methanotrophic bioreactors have the potential to convert recovered methane into valuable products such as single-cell protein and bioplastics, as well as produce methanotrophic biomass that can be incorporated into landfill cover soil. Recent advancements in anaerobic secondary treatment of wastewater in temperate climates have indicated a net energy-positive operation.
Increased disease transmission is possible due to climate change and its related heat, flooding, deteriorated water quality, and disease vectors, especially in densely populated areas. Further research is needed to find out how efficient and comprehensive pathogen surveillance can be in handling disease outbreaks, including those caused by climate change.
Microbes are more opportunistic and adaptable than humans. Despite the fact that the overwhelming majority of viruses, bacteria and fungi do not cause disease, climate change is causing all organisms to migrate, resulting in unparalleled interactions between hosts, vectors, and microbes.
Warmer temperatures, droughts, and extreme weather have resulted in the emergence of new pathogens, such as Candida auris, that have become thermally adapted for growth in humans.
Warmer temperatures affect the density of airborne microbes and can hasten their long-distance transport. Elevated temperature and environmental stresses can alter human and animal physiology and pathogen defenses. Increased monitoring and investigations of pathogen responses to climate change and their effects on host-pathogen interactions will be critical to reducing climate change impacts.
The advancement of pathogen surveillance could aid public understanding of the connection between climate change and health threats. According to a recent report by the IPCC, climate-sensitive aquatic pathogens such as Vibrio spp. have elevated regional risks of water and foodborne disease.
People may be able to recognize microbial threats if education and research are based on a more holistic, “One Health” way of thinking.
Metagenomics and other omics technologies hold great potential to offer the required data inputs to inform climate models and pathogen surveillance efforts in light of the global warming scenarios.
Microbial communities’ metabolic dynamics and phenotypic properties are largely unexplored. Recognizing how microbes interact across species, actively cycle nutrients, and respond to disturbances (extreme weather events or fires) could help quantify metabolically relevant characteristics.
New molecular assays for measuring metabolic rates in situ and with high-throughput resolution could revolutionize microbiome monitoring. Likewise, recent experimental and statistical approaches to link genomes from microbial isolates to community-level metabolic phenotypes could be used to deduce dynamic processes from metagenome data.
The crucial necessity of data infrastructure and support for open data sharing practices is critical to advancing innovative tools. Creating a framework for coordinated microbiome protocols and data-sharing infrastructure, similar to what was created as part of the international, multidisciplinary Tara Oceans project, would be an immediate step forward.
Integrating microbes into climate models will be aided by a data-driven approach and a robust shared data infrastructure, resulting in enhanced climate projections and possible new ways to mitigate that will inevitably benefit society.
Conclusion
There is a plethora of evidence that microbes play a role in climate change. Microbes are still significant players in current atmospheric changes, including oceanic, terrestrial, and urban settings.
As it is possible to enhance changes in microbial activities in some or several more environments to consume more and generate fewer gases that contribute to the warming of the atmosphere, the microbial world could become a critical ally in efforts to mitigate the consequences of human GHG emissions.
Further research and collaboration across disciplines are needed to address complex issues on the trio of relationships between climate change, microbes, and human well-being. The research community requires resourceful research networks and infrastructures, innovative tools, interconnected data and framework, and embedded climate models to enhance understanding.
Efforts to inform policies and educate the public must be coordinated to raise awareness and gain support. As a result, microbiologists must step up their efforts on various fronts to guarantee that all discussions about climate change involve microbial life’s contributions to these processes.
Journal Reference:
Tiedje, J. M., Bruns, M. A., Casadevall, A., Criddle, C. S., Eloe-Fadrosh, E., Karl, D. M., Nguyen, N. K., Zhou, J. (2022) Microbes and Climate Change: a Research Prospectus for the Future. mBio. Available Online: https://journals.asm.org/doi/10.1128/mbio.00800-22.
References and Further Reading
- IPCC. Climate change 2022: impacts, adaptation, and vulnerability. Contribution of Working Group II to the Sixth Assessment Report of the Intergovernmental Panel on Climate Change, in press. Cambridge University Press, Cambridge, United Kingdom
- Crowther T. W., et al. (2019) The global soil community and its influence on biogeochemistry. Science, 365:772. doi.org/10.1126/science.aav0550
- Guo X., et al. (2018) Climate warming leads to divergent succession of grassland microbial communities. Nature Climate Change, 8, pp. 813–818. doi.org/10.1038/s41558-018-0254-2.
- Guo X., et al. (2019) Climate warming accelerates temporal scaling of grassland soil microbial biodiversity. Nature Ecology & Evolution, 3, pp. 612–619. doi.org/10.1038/s41559-019-0848-8.
- Yuan M. M., et al. (2021) Climate warming enhances microbial network complexity and stability. Nature Climate Change, 11, pp. 343–348. doi.org/10.1038/s41558-021-00989-9.
- Guo X., et al. (2020) Gene-informed decomposition model predicts lower soil carbon loss due to persistent microbial adaptation to warming. Nature Communications, 11, p. 4897. https://www.nature.com/articles/s41467-020-18706-z#citeas
- Luo Y., et al. (2001) Acclimatization of soil respiration to warming in a tall grass prairie. Nature, 413, pp. 622–625. doi.org/10.1038/35098065.
- Zhou J., et al. (2012) Microbial mediation of carbon-cycle feedbacks to climate warming. Nature Climate Change, 2, pp. 106–110. doi.org/10.1038/nclimate1331.
- Crowther, T. W., et al. (2016) Quantifying global soil carbon losses in response to warming. Nature, 540, pp. 104–108. doi.org/10.1038/nature20150.
- Carey, J. C., et al. (2016) Temperature response of soil respiration largely unaltered with experimental warming. Proceedings of the National Academy of Sciences USA, 113, pp. 13797–13802. doi.org/10.1073/pnas.1605365113.
- National Academies of Sciences, Engineering, and Medicine. 2021. Exploring a dynamic soil information system: proceedings of a workshop. The National Academies Press, Washington, DC. doi.org/10.17226/26170.
- Borer E. T., et al. (2014) Finding generality in ecology: a model for globally distributed experiments. Methods in Ecology and Evolution, 5, pp. 65–73. doi.org/10.1111/2041-210X.12125.
- Gao Q., et al. (2020) Stimulation of soil respiration by elevated CO2 is enhanced under nitrogen limitation in a decade-long grassland study. Proceedings of the National Academy of Sciences USA, 117, pp. 33317–33324. https://doi.org/10.1073/pnas.2002780117.
- Longhurst A (2006) Ecological geography of the sea, 2nd ed. Academic Press, New York, NY.
- Hagstrom G I & Levin S A (2017) Marine ecosystems as complex adaptive systems: emergent patterns, critical transitions, and public goods. Ecosystems, 20, pp. 458–476. doi.org/10.1007/s10021-017-0114-3.
- Gruber N (2011) Warming up, turning sour, losing breath: ocean biogeochemistry under global change. Philosophical Transactions of the Royal Society A, Mathematical, Physical and Engineering Sciences, 369, pp. 1980–1996. doi.org/10.1098/rsta.2011.0003.
- Oliver, E. C. J., et al. (2018) Longer and more frequent marine heatwaves over the past century. Nature Communications, 9, p. 1324. doi.org/10.1038/s41467-018-03732-9.
- Frölicher, T. L., et al. (2018) Marine heatwaves under global warming. Nature, 560, pp. 360–364. doi.org/10.1038/s41586-018-0383-9.
- Solomon S., et al. (2009) Irreversible climate change due to carbon dioxide emissions. Proceedings of the National Academy of Sciences USA, 106, pp. 1704–1709. doi.org/10.1073/pnas.0812721106.
- Karl, D. M., et al. (2003) Temporal studies of biogeochemical processes determined from ocean time-series observations during the JGOFS era. In Fasham MJR (ed), Ocean biogeochemistry: the role of the ocean carbon cycle in global change, p 239–267. Springer, New York, NY.
- Boyd. P. W., et al. (2018) Experimental strategies to assess the biological ramifications of multiple drivers of global ocean change—a review. Global Change Biology, 24, pp. 2239–2261. doi.org/10.1111/gcb.14102.
- Hobbs, R. J., et al. (2011) Intervention ecology: applying ecological science in the twenty-first century. Bioscience, 61, pp. 442–450. doi.org/10.1525/bio.2011.61.6.6.
- National Academies of Sciences, Engineering, and Medicine. 2021. A research strategy for ocean-based carbon dioxide removal and sequestration. The National Academies Press, Washington, DC. doi.org/10.17226/26278.
- El Abbadi S H & Criddle, C S (2019) Engineering the dark food chain. Environmental Science & Technology, 53, pp. 2273–2287. doi.org/10.1021/acs.est.8b04038.
- Berendes, D. M., et al. (2018) Estimation of global recoverable human and animal faecal biomass. Nature Sustainability, 1, pp. 679–685. doi.org/10.1038/s41893-018-0167-0.
- Shin C., et al. (2021) Temperate-climate energy-positive anaerobic secondary treatment of domestic wastewater achieved at pilot-scale. Water Research, 204, p. 117598. doi.org/10.1016/j.watres.2021.117598.
- Jana P., et al. (2011) Co-production of graphene sheets and hydrogen by decomposition of methane using cobalt based catalysts. Energy & Environmental Science, 4, pp. 778–783. doi.org/10.1039/C0EE00490A.
- Woo, S. G., et al. (2022) Phylogenetic diversity of NO reductases, new tools for nor monitoring, and insights into N2O production in natural and engineered environments. Frontiers of Environmental Science & Engineering, 16, p. 127. doi.org/10.1007/s11783-022-1562-3.
- Shan J., et al. (2021) Beyond denitrification: the role of microbial diversity in controlling nitrous oxide reduction and soil nitrous oxide emissions. Global Change Biology, 27, pp. 2669–2683. doi.org/10.1111/gcb.15545.
- Fouladkhah A. C., et al. (2020) The threat of antibiotic resistance in changing climate. Microorganisms, 8, p. 748. doi.org/10.3390%2Fmicroorganisms8050748.
- Robert V., et al. (2015) Distribution and impact of yeast thermal tolerance permissive for mammalian infection. BMC Biology, 13, p. 18. doi.org/10.1186/s12915-015-0127-3.
- Nnadi N E & Carter D A (2021) Climate change and the emergence of fungal pathogens. PLoS Pathogens, 17, p. e1009503. doi.org/10.1371/journal.ppat.1009503
- Drautz-Moses, D. I., et al. (2022) Vertical stratification of the air microbiome in the lower troposphere. Proceedings of the National Academy of Sciences USA, 119, p. e2117293119. doi.org/10.1073/pnas.2117293119.
- Morawska, L. P., et al. (2021) Diversity of bet-hedging strategies in microbial communities—recent cases and insights. WIRES Mechanisms of Disease, 14, p. e1544. doi.org/10.1002/wsbm.1544.
- Cavicchioli, R., et al. (2019) Scientists' warning to humanity: microorganisms and climate change. Nature Reviews Microbiology, 17, pp. 569–586. doi.org/10.1038/s41579-019-0222-5.
- Mackenzie, J S & Jeggo M (2019) The One Health approach: why is it so important? Tropical Medicine and Infectious Disease, 4, p. 88. doi.org/10.3390%2Ftropicalmed4020088.
- American Academy of Microbiology (2011) Incorporating microbial processes into climate models: this report is based on a colloquium convened by the American Academy of Microbiology, February 21–23, 2011, in Dallas, TX. American Society for Microbiology, Washington, DC. https://www.ncbi.nlm.nih.gov/books/NBK561255/
- Almeida, A., et al. (2021) A unified catalog of 204,938 reference genomes from the human gut microbiome. Nature Biotechnology, 39, pp. 105–114. doi.org/10.1038/s41587-020-0603-3.
- Nayfach, S., et al. (2021) A genomic catalog of Earth’s microbiomes. Nature Biotechnology, 39, pp. 499–509. doi.org/10.1038/s41587-020-0718-6.
- Gowda, K., et al. (2022) Genomic structure predicts metabolite dynamics in microbial communities. Cell, 185, p. 530–546.e25. doi.org/10.1016/j.cell.2021.12.036.
- Prosser, J I & Martiny, J B (2020). Conceptual challenges in microbial community ecology. Philosophical Transactions of the Royal Society B, 375, p. 20190241. doi.org/10.1098/rstb.2019.0241.
- Bar-On Y M., et al. (2018) The biomass distribution on Earth. Proceedings of the National Academy of Sciences USA, 115, pp. 6506–6511. doi.org/10.1073/pnas.1711842115.
- Neubauer, S C & Megonigal, J P (2015). Moving beyond global warming potentials to quantify the climatic role of ecosystems, Ecosystems, 18, pp. 1000–1013. doi.org/10.1007/s10021-015-9879-4.
- American Academy of Microbiology (2022) Microbes & Climate Change: Science, People, and Impacts: Report on an American Academy of Microbiology (Academy) Virtual Colloquium held on 5 November 2021: American Society for Microbiology, Washington, DC. https://www.ncbi.nlm.nih.gov/books/NBK560256/.