Electric vehicles are viewed as a solution to the era of energy conservation and environmental preservation since they solve the problems of energy loss and pollution. At low temperatures, however, lithium-ion batteries have certain limitations, such as weak electrolyte ionic conductivity, slow chemical reaction rate, and poor conductivity of the SEI coating on the surface of negative graphite particles.
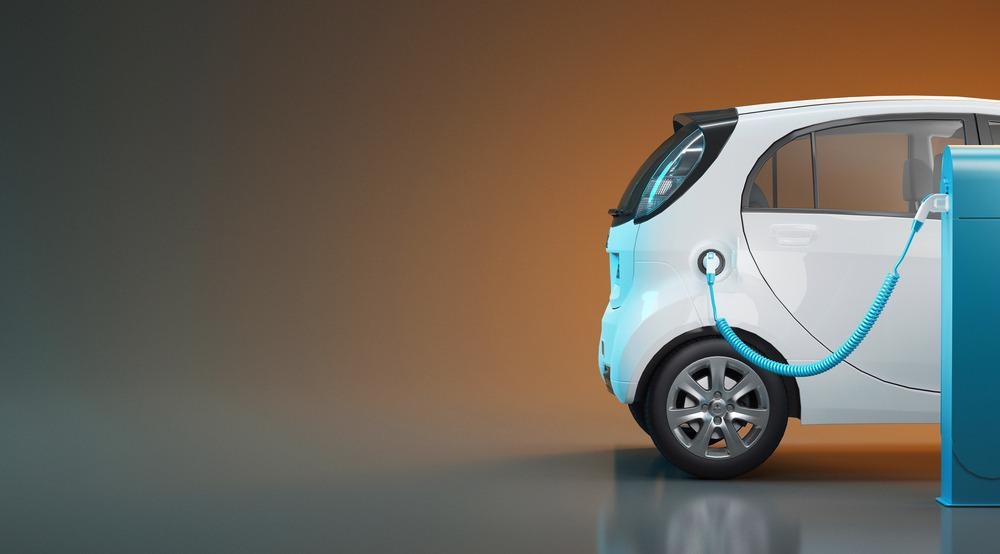
Image Credit: ALDECA studio/Shutterstock.com
At low temperatures, the diffusion coefficient of lithium-ion in anode graphite particles is particularly low, resulting in slow charging.
It is also simple for lithium-ions to concentrate on a negative surface during charging and produce lithium metal at low temperatures, causing irreversible damage to the battery after a lengthy period of lithium metal dendrite development.
As a result, it is important to look into a new technique for dealing with the aforementioned challenges in the case of low-temperature batteries.
As a way of charging at low temperatures, warm air heating, liquid heating, film heating, and circulating high-temperature gas heating have all been examined.
Internal self-heating, internal resistance heating, convection heating, pulse heating, and alternating current (AC) excitation heating are some examples of internal heating systems.
Warm air heating is easy and inexpensive, although it is inefficient compared to liquid heating. The liquid heating method is comparable to circulating high-temperature gas heating. However, due to the greater liquid thermal conductivity, the liquid heating effect is superior to gas heating.
The heat exchange between the liquid and the batteries warms the batteries in the liquid heating technique. Oil, glycol, and water are the most often employed heat transfer media. This approach involves submerging the batteries in liquid or a pipeline around the battery.
However, this approach necessitates a highly airtight battery or battery box, which increases the challenge of battery construction.
Methodology
For lithium-ion batteries to charge fast in low-temperature situations, they must have a short charging time and a rapid temperature increase without losing battery life or safety.
To prevent lithium plating, the negative area of lithium-ion batteries should have a substantial solid-liquid potential difference during charging, but the overall polarization voltage should be low.
Furthermore, the upper and lower cutoff voltages must be higher than the voltage at the lithium-ion battery’s two ends.
The effectiveness of the AC excitation charging method was evaluated using the charging speed and temperature increase speed of the LG INR18650HG2 battery. Table 1 lists the features of the test battery.
Table 1. The parameters of the test battery. Source: Guo, et al., 2022
Brand Model |
LG INR18650HG2 |
Standard capacity |
3000 mAh |
Rated voltage |
3.6 V |
Charging voltage |
4.2 ± 0.05 V |
Battery charging |
Standard 1500 mA; cutoff current 50 mA(cc-cv) |
Fast charge |
4000 mA, cutoff current 100 mA(cc-cv) |
Maximum continuous discharge current |
20 A |
Discharge cut-off voltage |
2.5 V |
Essential resistance (AC 1 KHz) |
≤20 mΩ, with our PTC |
Figures 1 and 2 show the experimental devices in action. The electricity was provided via a thermal chamber and an inverter.
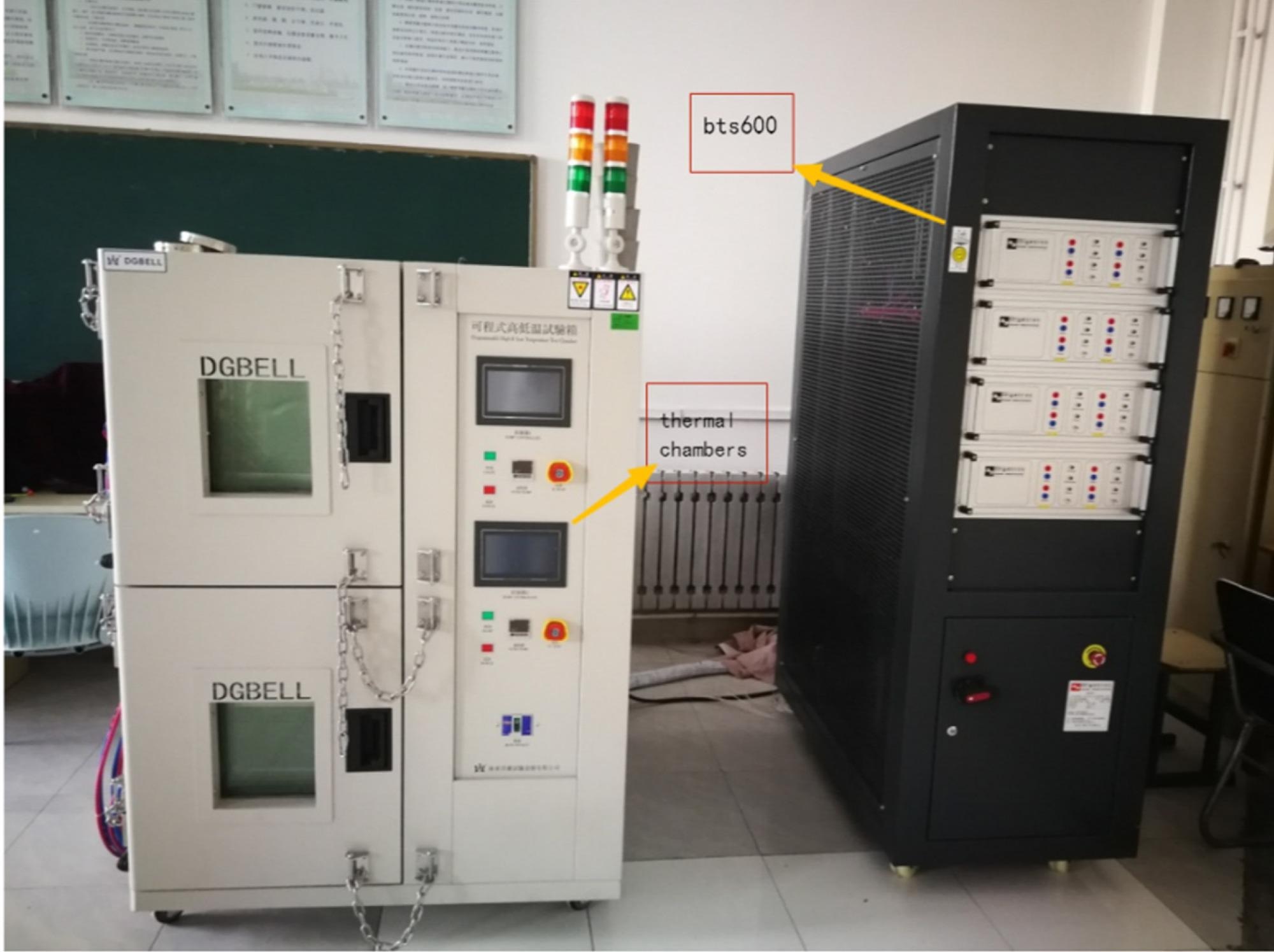
Figure 1. The BTS600 and thermal chamber. Image Credit: Guo, et al., 2022
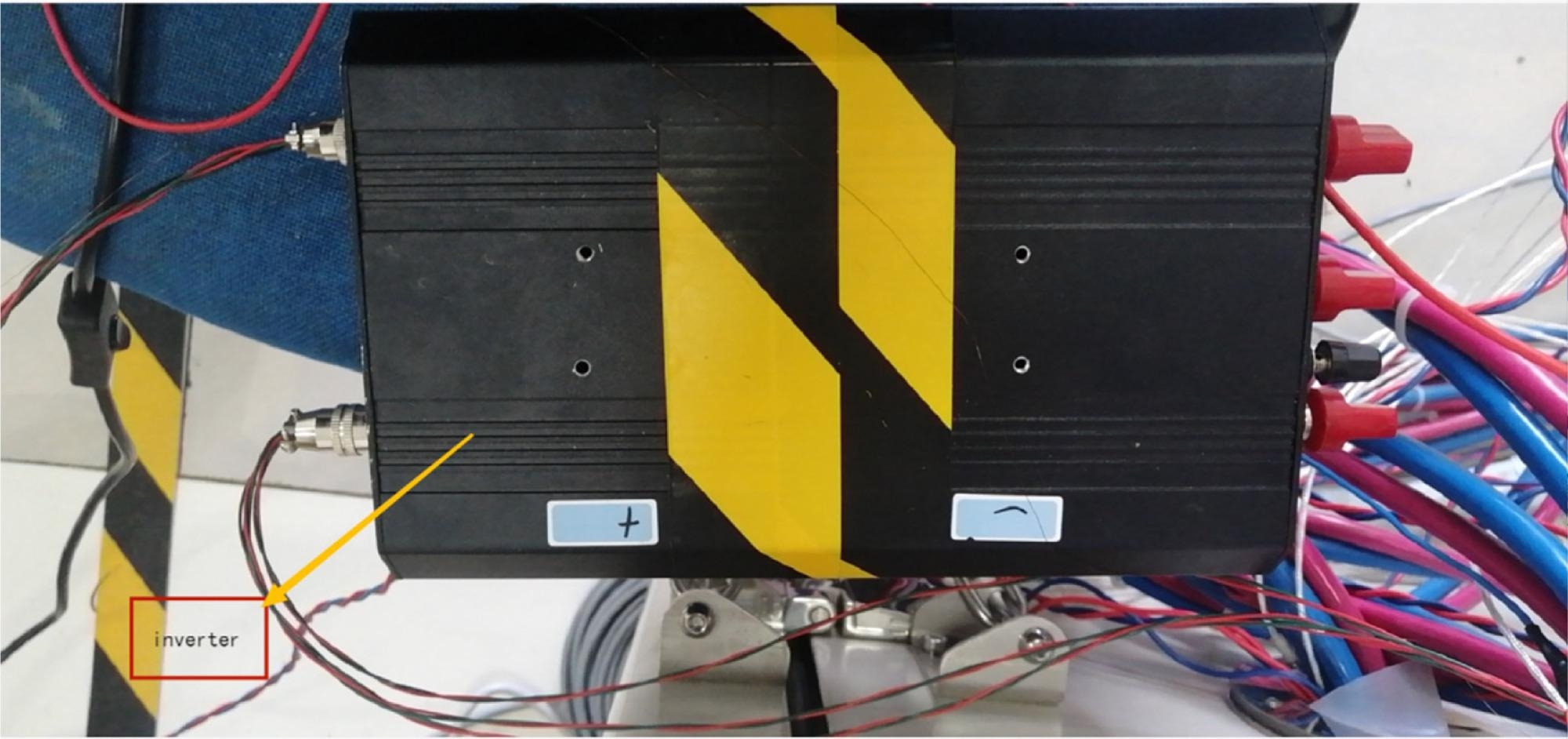
Figure 2. The AC inverter. Image Credit: Guo, et al., 2022
The BTS 600 was used to calibrate the battery’s capacity using uniform current and constant voltage charge and discharge. An inverter provided the alternating current electricity. A computer was utilized to regulate the charging and discharging of the batteries and to collect the data using a technique.
Temperature setting, capacity testing, SOC calibration, and charging tests at low temperatures were all part of the test. During the charging and discharging processes, numerous parameters were monitored and recorded.
4.2 V and 2.5 V were the cut-off voltages during the charging and discharging periods, respectively. A temperature sensor was installed in the center of the battery surface to detect temperature changes. The voltage and current were measured by sensors and reported in real-time by the computer.
The battery was charged using an AC incentive approach at 0 °C and 5 °C.
The battery must be kept static for more than two hours in each environment to guarantee that the battery temperature in the laboratory approaches the exterior ambient temperature.
Using the 0% SOC battery as an example, as seen in Figure 3, varied temperature increase rates were observed at various external temperatures. Firstly, the battery temperature rose quicker at 5 °C than at 0 °C. Secondly, when the temperature climbed, the rate of rising remained steady.
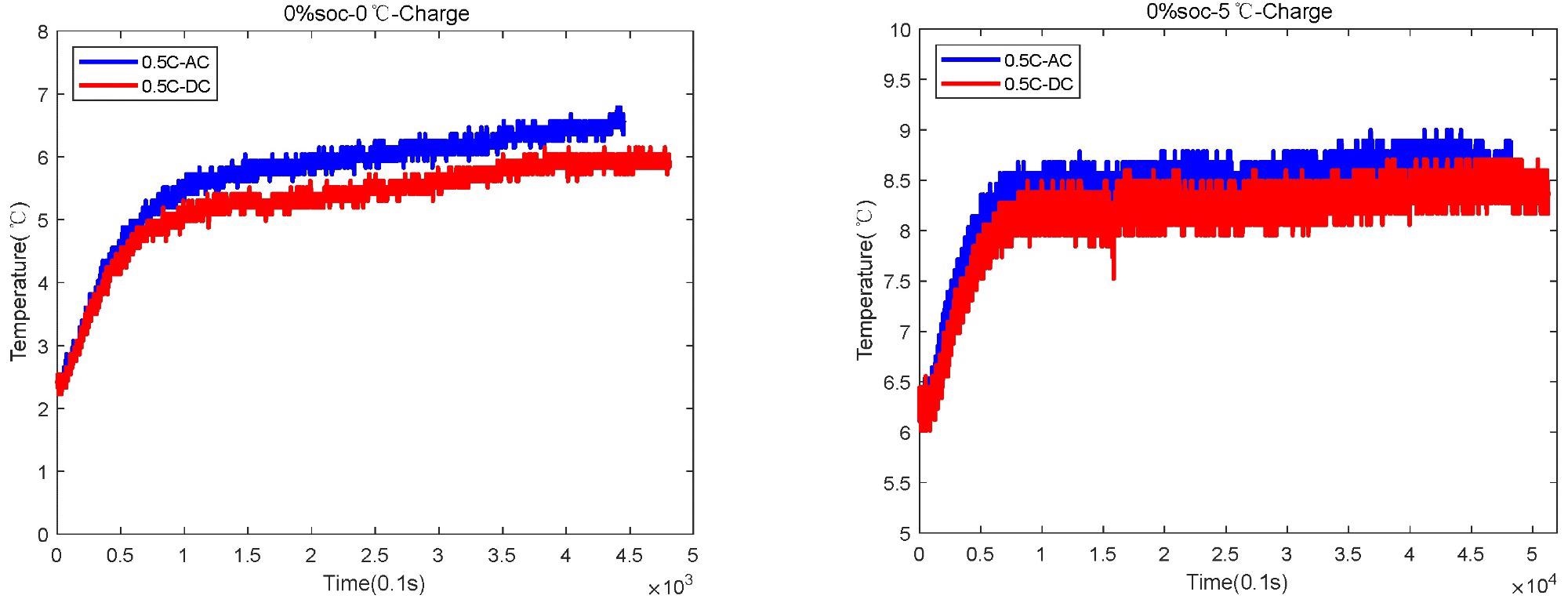
Figure 3. The temperature in the case of charging starting at 0% SOC at 0 °C and 5 °C respectively. Image Credit: Guo, et al., 2022
Finally, in the 5 °C ambient condition, the final battery temperature was greater than the 0 °C battery temperature.
Figure 4 shows that the battery voltage increased significantly at the start of charging. As charging progressed, the rate of growth grew progressively. When charging began at 0% SOC, the 5 °C DC charging time was 200 seconds quicker than the 0 °CDC charging time, and the 5 °C AC charging time was 100 seconds faster than the 0 °C DC charging time.
This suggests that the AC charging approach outperformed the DC charging strategy.
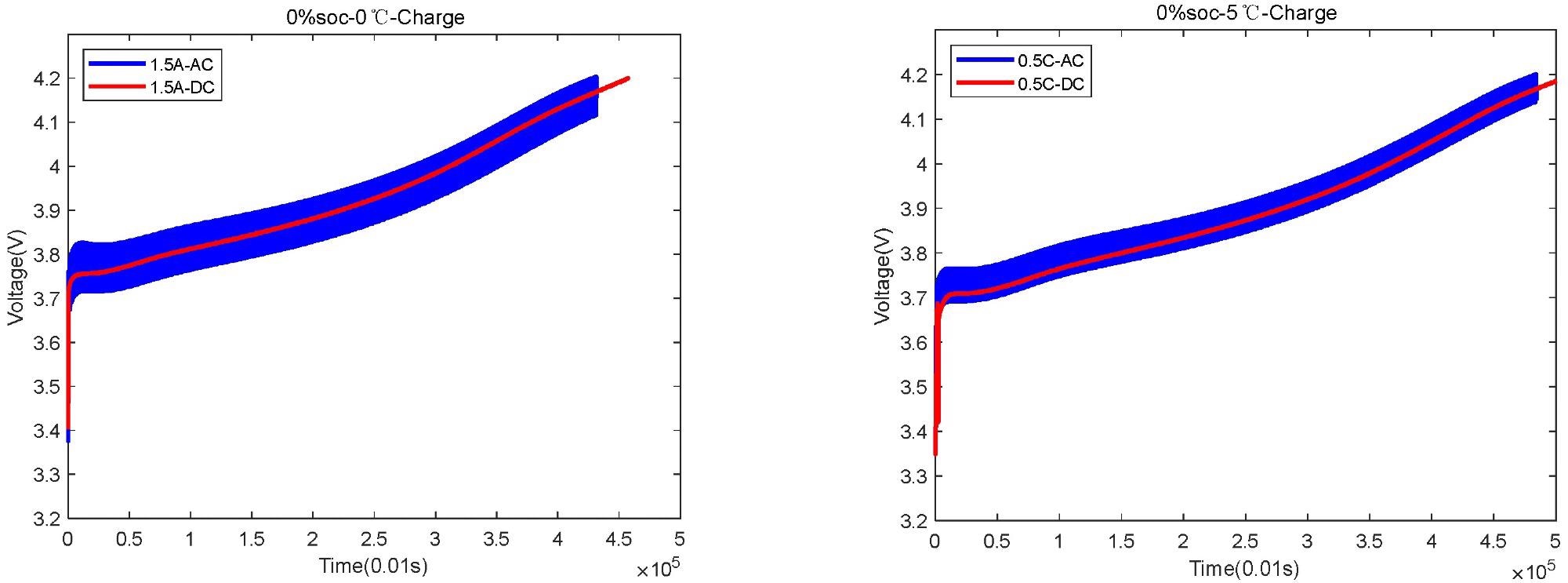
Figure 4. The voltage changes in the case of charging starting at 0% SOC at 0 °C and 5 °C, respectively. Image Credit: Guo, et al., 2022
Results
The battery’s starting capacity was 25%, as indicated in Figure 5, and it was charged in a 5 °C external environment. The temperature change graph on the left shows that the battery temperature rises significantly during the initial charging stage.
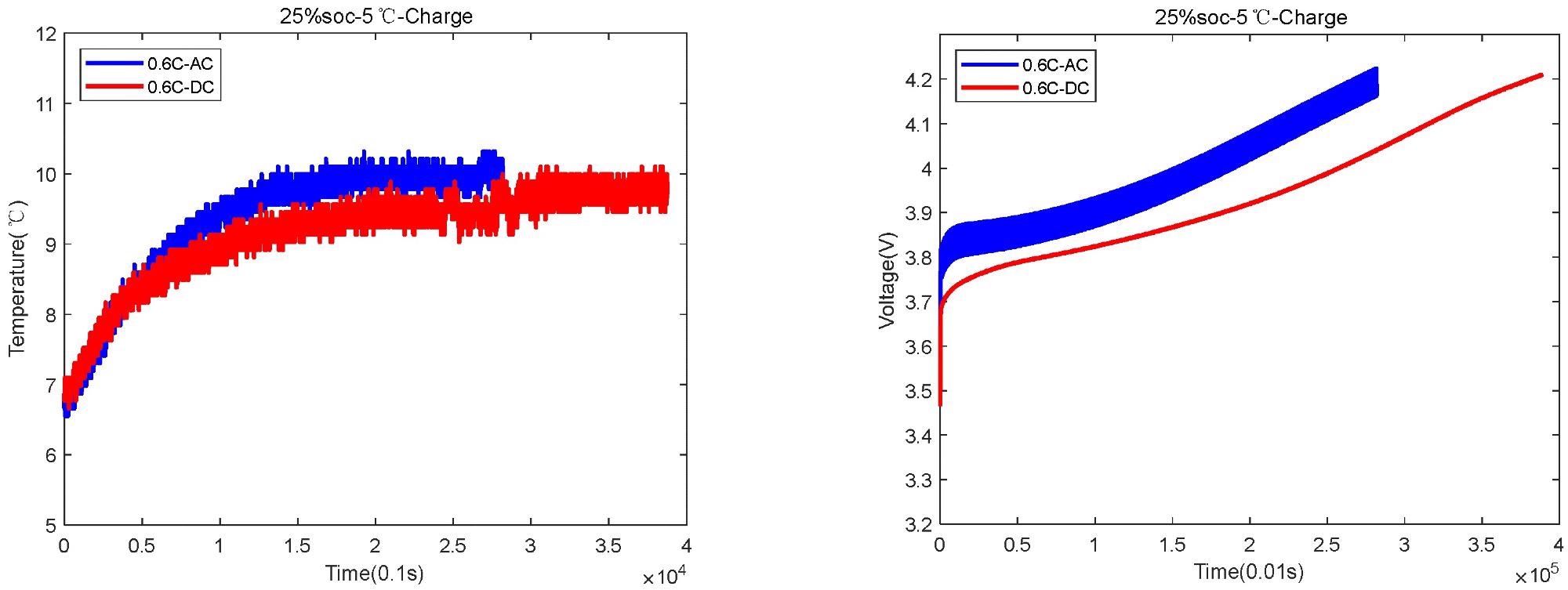
Figure 5. The temperature and voltage changes in the case of charging starting at 25% SOC and 5 °C. Image Credit: Guo, et al., 2022
It is clear that the voltage increased rapidly at the start of charging. On average, the voltage curve of AC charging was higher than that of DC charging. The charging pace was slow in the beginning, the voltage grew slowly, and the curve was flat.
In contrast, the charging rate increased and the slope of the curve increased in the latter charging phases, which might be attributed to a rise in temperature and a decrease in the internal resistance of the battery.
The temperature and voltage variations in Figures 6, 7, and 8 are identical to those in Figure 5.
When the initial battery capacity is 25% and the outside temperature is 0 °C, the AC charging time is 1300 seconds, and the temperature rises by 8 °C, respectively, as illustrated in Figure 6.
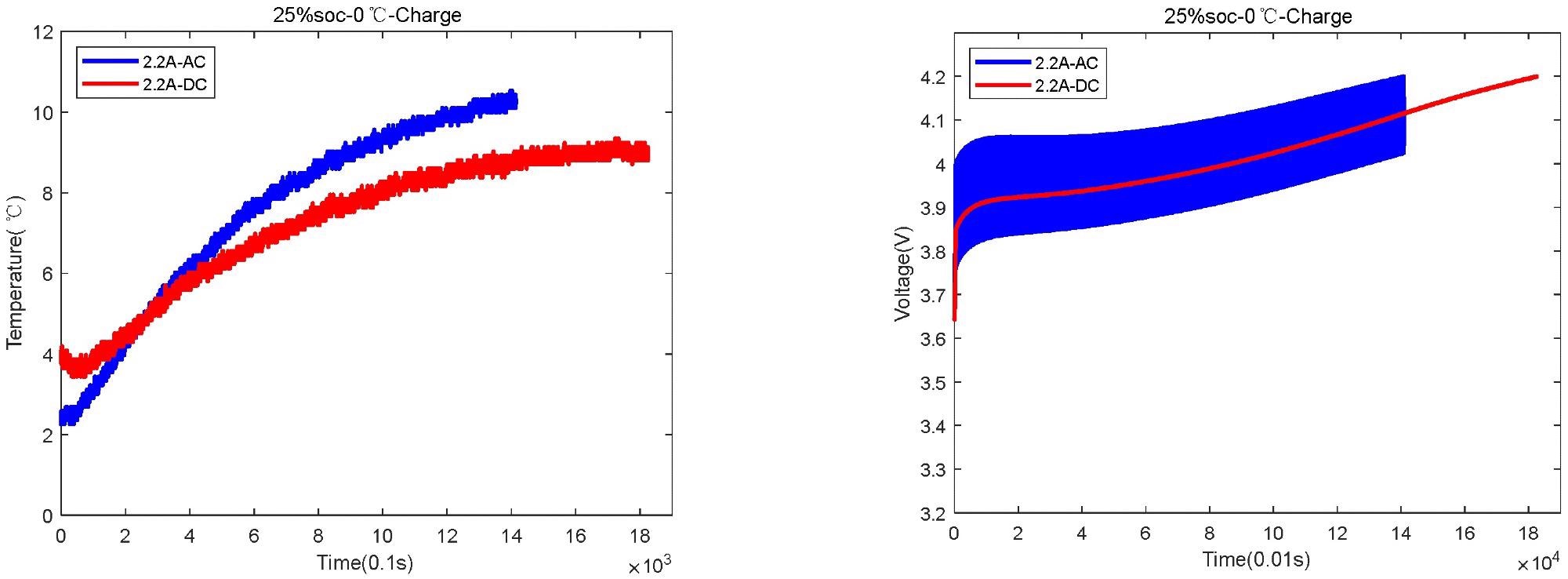
Figure 6. 0 °C and 25% SOC temperature and voltage change diagram. Image Credit: Guo, et al., 2022
Figure 7 shows that when the initial battery capacity was 50%, the external temperature was 5 °C, and the charging current was 0.8 c, the AC full charging time was 1300 seconds and the DC full charging time was 1500 seconds. The charging speed has been boosted by 13%.
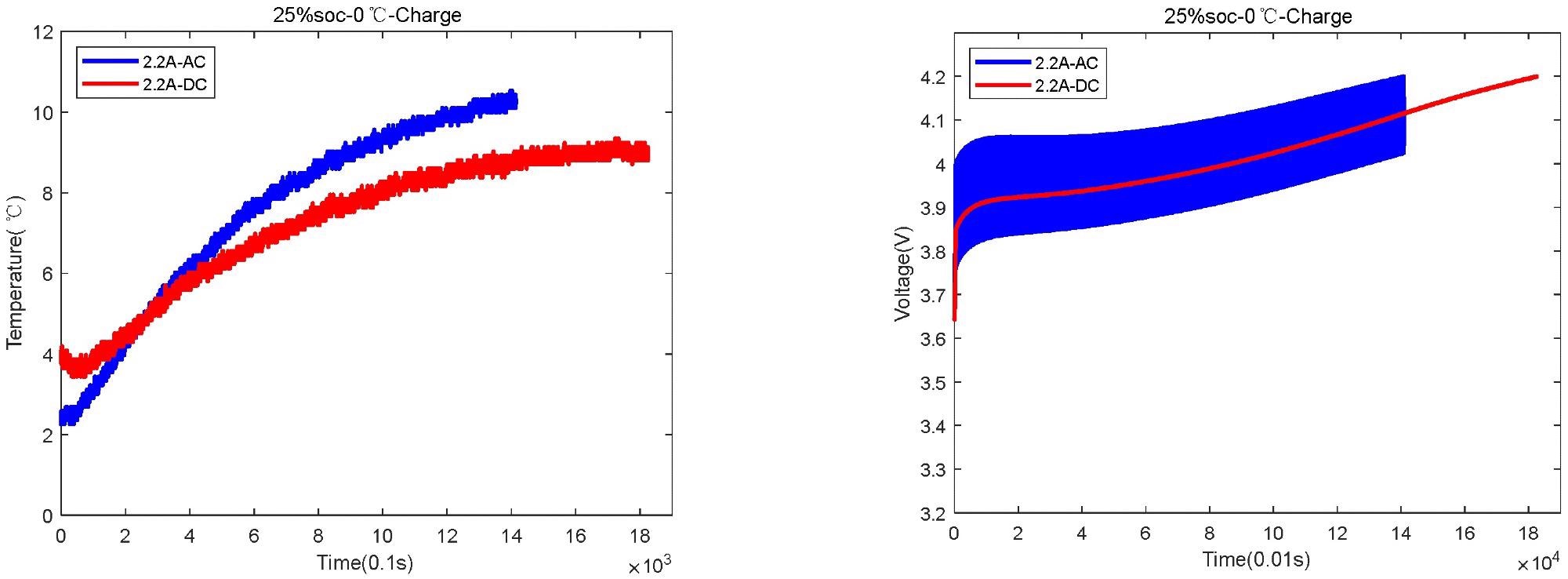
Figure 7. 5 °C and 50% SOC Temperature and voltage change diagram. Image Credit: Guo, et al., 2022
The AC full charging time was 4300 seconds and the DC full charging time was 4900 seconds when the initial battery capacity was 0%, the battery exterior temperature was set to 0 °C, and the current user was set to 0.5 c, as shown in Figure 8. The charging speed has already been boosted by 12%.
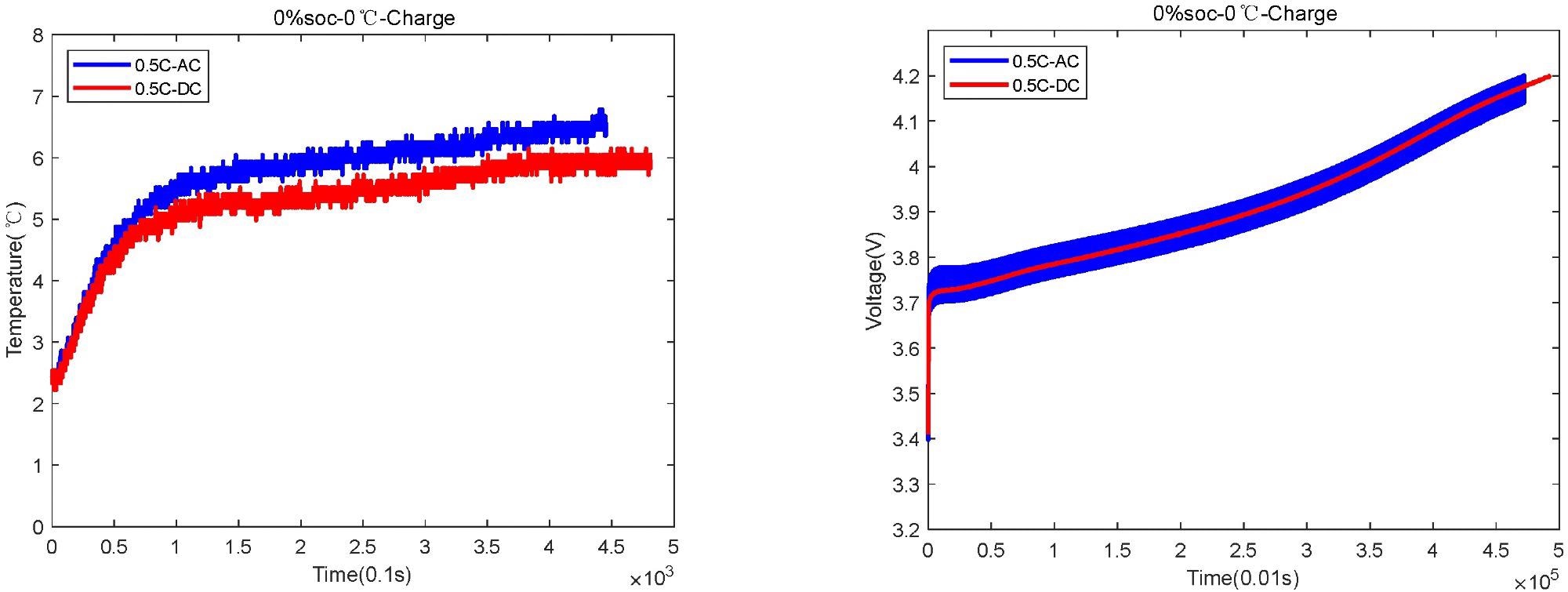
Figure 8. 0 °C and 0% SOC temperature and voltage change diagram. Image Credit: Guo, et al., 2022
Table 2 shows the particular temperature changes as well as the charging time.
Table 2. Temperature and time. Source: Guo, et al., 2022
|
Charging Time |
Temperature Variation |
0%SOC-0 °C |
4300 s AC and 4800 s DC |
AC temperature rose by 5 °C, and DC temperature rose by 3.5 °C |
0%SOC-5 °C |
4700 s for AC and 5200 s for DC |
AC temperature rose by 3 °C, and DC temperature rose by 2.5 °C |
25%SOC-0 °C |
1300 s for AC and 1800 s for DC |
AC temperature rose by 8.5 °C, and DC temperature rose by 5 °C |
25%SOC-5 °C |
2700 s for AC and 3800 s for DC |
AC temperature rose by 3.5 °C, and DC temperature rose by 3 °C |
50%SOC-5 °C |
1400 s for AC and 1500 s for DC |
AC temperature rose by 6 °C, and DC temperature rose by 4.5 °C |
Conclusions
The AC excitation charging method was used to study a low-temperature rapid charging strategy in the study. To monitor temperature change, voltage change, and charge power, the system performed DC and AC charging and discharging tests with various starting SOCs at various temperatures.
The AC excitation charging approach is 20% quicker than the CCCV method. Compared to the CCCV approach, the AC excitation charging strategy exhibits a variable voltage rise. As a result, the temperature increases quicker than with the CCCV approach, with an average temperature increase of 2–4 °C.
Therefore, the suggested charging technique, which is superior to the current CCCV approach, can greatly cut charging time at a reasonable temperature rise.
The AC excitation charging strategy might be employed for quick charging of electric automobiles in low-temperature conditions in the future.
Journal Reference:
Guo, S., Han, Z., Wei, J., Guo, S. and Ma, L. (2022) A Novel DC-AC Fast Charging Technology for Lithium-Ion Power Battery at Low-Temperatures. Sustainability. 14(11), 6544 Available Online: https://www.mdpi.com/2071-1050/14/11/6544/htm
References and Further Reading
- Bresser, D., et al. (2018) Perspectives of automotive battery R&D in China, Germany, Japan, and the USA. Journal of Power Sources, 382, pp. 176–178. doi.org/10.1016/j.jpowsour.2018.02.039.
- Esfandyari, M., et al. (2019) A new approach to consider the influence of aging state on Lithium-ion battery state of power estimation for hybrid electric vehicle. Energy, 176, pp. 505–520. doi.org/10.1016/j.energy.2019.03.176
- Li, S., et al. (2021) Energy management for hybrid energy storage system in electric vehicle: A cyber-physical system perspective. Energy, 230, p. 120890. doi.org/10.1016/j.energy.2021.120890.
- Xiong, R, et al. (2020) Lithium-ion battery aging mechanisms and diagnosis method for automotive appli-cations: Recent advances and perspectives. Renewable and Sustainable Energy Reviews, 131, p. 110048. doi.org/10.1016/j.rser.2020.110048.
- Yang, R., et al. (2020) Extreme Learning Machine Based Thermal Model for Lithi-um-ion Batteries of Electric Vehicles under External Short Circuit. Engineering, 7, pp. 395–405. doi.org/10.1016/j.eng.2020.08.015.
- Xiong, R., et al. (2020) Research progress, challenges and prospects of fault diagnosis on battery system of electric vehicles. Applied Energy, 279, p. 115855. doi.org/10.1016/j.apenergy.2020.115855.
- Ji, Y., et al. (2013) Li-Ion Cell Operation at Low Temperatures. Journal of The Electrochemical Society, 160, pp. A636–A649.
- Li, J., et al. (2012) Limiting factors for low-temperature performance of electrolytes in LiFePO 4/Li and graphite/Li half cells. Electrochimica Acta, 59, pp. 69–74. doi.org/10.1016/j.electacta.2011.10.041.
- Abraham, D. P., et al. (2008) Investigating the Low-Temperature Impedance Increase of Lithium-Ion Cells. Journal of the Electrochemical Society, 155, pp. A41–A47.
- Zhang, S., et al. (2002) A new approach toward improved low temperature performance of Li-ion battery. Electrochemistry Communications, 4, pp. 928–932. doi.org/10.1016/S1388-2481(02)00490-3.
- Zhang, S., et al. (2005) An inorganic composite membrane as the separator of Li-ion batteries. Journal Power Sources, 140, pp. 361–364. doi.org/10.1016/j.jpowsour.2004.07.034.
- Zhang, S., et al. (2002) Low temperature performance of graphite electrode in Li-ion cells. Electrochimuica Acta, 48, pp. 241–246. doi.org/10.1016/S0013-4686(02)00620-5.
- Qiao, Y., et al. (2012) The low and high temperature electrochemical performances of Li3V2(PO4)3/C cathode ma-terial for Li-ion batteries. Journal of Power Sources, 199, pp. 287–292. doi.org/10.1016/j.jpowsour.2011.10.054.
- Zhang, S., et al. (2004) Electrochemical impedance study on the low temperature of Li-ion batteries. Electrochimuica Acta, 49, pp. 1057–1061. doi.org/10.1016/j.electacta.2003.10.016.
- Zhang, S., et al. (2003) The low temperature performance of Li-ion batteries. Journal of Power Sources, 115, pp. 137–140. doi.org/10.1016/S0378-7753(02)00618-3.
- Fan, J & Tan, S (2006) Studies on Charging Lithium-Ion Cells at Low Temperatures. Journal of The Electrochemical Society, 153, pp. A1081–A1092. doi.org/10.1149/1.2190029.
- Bitzer, B & Gruhle, A (2014) A new method for detecting lithium plating by measuring the cell thickness. Journal of Power Sources, 262, pp. 297–302. doi.org/10.1016/j.jpowsour.2014.03.142.
- Legrand, N., et al. (2014) Physical characterization of the charging process of a Li-ion battery and predic-tion of Li plating by electrochemical modeling.Journal of Power Sources,245, pp. 208–216. doi.org/10.1016/j.jpowsour.2013.06.130.
- Uhlmann, C., et al. (2015) In situ detection of lithium metal plating on graphite in experimental cells. Journal of Power Sources, 279, pp. 428–438. doi.org/10.1016/j.jpowsour.2015.01.046.
- Zinth, V., et al. (2014) Lithium plating in lithium-ion batteries at sub-ambient temperatures investigated by in situ neutron diffraction. Journal of Power Sources, 271, pp. 152–159. doi.org/10.1016/j.jpowsour.2014.07.168.
- Cho, H. M., et al. (2012) A study on time-dependent low temperature power performance of a lithium-ion battery. Journal of Power Sources, 198, 273–280. doi.org/10.1016/j.jpowsour.2011.09.111.
- Zhang, S., et al. (2006) Charge and discharge characteristics of a commercial LiCoO2-based 18650 Li-ion battery. Journal of Power Sources, 160, pp. 1403–1409. doi.org/10.1016/j.jpowsour.2006.03.037.
- Fan, J (2003) On the discharge capability and its limiting factors of commercial 18650 Li-ion cell at low temperatures. Journal of Power Sources, 117, pp. 170–178. doi.org/10.1016/S0378-7753(03)00354-9.
- Zhao, H., et al. (2009) Study on performance of low temperature type lithium ion battery. Chin. Journal of Power Sources, 1, p. 019.
- Harris, V & Miller, R L (1981) Vehicle Heating and Cooling System. U.S. Patent 4,280,330, 28 July.
- Song, H. S., et al. (2012) Experimental study on the effects of pre-heating a battery in a low-temperature environment. In Proceedings of the 2012 IEEE Vehicle Power and Propulsion Conference, Seoul, Korea, 9–12 October; pp. 1198–1201. doi.org/10.1109/VPPC.2012.6422509.
- Furman, G R (1948) Automotive Battery Heating System. U.S. Patent 2,440,369, 7 April.
- Malecek, E L (1998) Battery Heating Device and Method. U.S. Patent 5,731,568, 24 March.
- Godard, P & Prevot, C (1977) Method and Device for Charging and Heating at Low Temperature a Sealed Storage Cell Battery. U.S. Patent 4,025,861, 24 May.
- Kamenoff, R (2006) Self-Heating Battery That Automatically Adjusts Its Heat Setting. U.S. Patent 11,178,985, 19 January.
- Wilmer, K (1952) Battery Heater. U.S. Patent 2,611,853, 23 September.
- Mccall, D J (1999) Battery Warmer with Timer Switch. U.S. Patent 5,994,669, 30 November.
- Tian, J., et al. (2021) State-of-charge estimation of LiFePO4 batteries in electric vehicles: A deep-learning enabled approach. Applied Energy, 291, p. 116812. doi.org/10.1016/j.apenergy.2021.116812.
- Ashtiani, C N (2008) Heating of Batteries Using Reactive Power. U.S. Patent 7,382,102, 3 June.
- Ashtiani, C N & Stuart, T A (2005) Battery Self-Warming Mechanism Using the Inverter and the Battery Main Disconnect Circuitry. U.S. Patent 6,882,061, 19 April.
- Ashtiani, C N & Stuart, T A (2000) Efficient Resonant Self-Heating Battery Electric Circuit. U.S. Patent 6,072,301, 6 June.
- Carkner, S (2015) Self Heating Battery System. U.S. Patent 9,083,065, 14 July.
- Hande, A (2006) Internal battery temperature estimation using series battery resistance measurements during cold tempera-tures. Journal of Power Sources, 158, pp. 1039–1046. doi.org/10.1016/j.jpowsour.2005.11.027.
- Hande, A (2004) A high frequency inverter for cold temperature battery heating. In Proceedings of the 2004 IEEE Workshop on Computers in Power Electronics, Urbana, IL, US, 15–18 August; pp. 215–222. doi.org/10.1109/CIPE.2004.1428157.
- Hande, A & Stuart, T (2002) AC heating for EV/HEV batteries. In Proceedings of the Power Electronics in Transportation, Auburn Hills, MI, USA, 24–25 October; pp. 119–124. doi.org/10.1109/PET.2002.1185559.
- Vanderslice, W T Jr & Scafidi, C J (1994) Battery Heating System Using Internal Battery Resistance. U.S. Patent 5,362,942, 8 November.
- Wu, X., et al. (2017) Analysis of Low Temperature Preheating Effect Based on Battery Temperature-Rise Model. Energies, 10, p. 1121. doi.org/10.3390/en10081121.
- Ji, Y & Wang, C Y (2013) Heating strategies for Li-ion batteries operated from subzero temperatures. Electrochimica Acta, 107, pp. 664–674. doi.org/10.1016/j.electacta.2013.03.147.
- Wang, C. Y., et al. (2016) Lithium-ion battery structure that self-heats at low temperatures. Nature, 529, p. 515. doi.org/10.1038/nature16502.
- Vernardou, D., et al. (2016) Hydrothermal Growth of MnO2 at 95 °C as an Anode Material. International Journal of Thin Film Science and Technology, 5, pp. 121–127. doi.org/10.18576/ijtfst/050207.
- Al-Qrinawi, M. S., et al. (2021) Capacitance-voltage measurements of hetero-layer OLEDs treated by an electric field and thermal annealing. International Journal of Thin Film Science and Technology, 10, pp. 217–226.
- Elhadary, A. A., et al. (2021) Studying the Effect of the Dielectric Barrier Discharge Non-thermal Plasma on Colon Cancer Cell line. International Journal of Thin Film Science and Technology, 10, pp. 161–168.
- Joshi, V & Kapoor, M (2021) A Novel Technique for Numerical Approximation of 2 Dimensional Non-Linear Coupled Burgers’ Equations Using Uniform Algebraic Hyperbolic (UAH) Tension B-Spline Based Differential Quadrature Method. Applied Mathematics & Information Sciences, 15, pp. 217–239.
- Von Lüders, C., et al. (2017) Lithium plating in lithium-ion batteries inves-tigated by voltage relaxation and in situ neutron diffraction. Journal of Power Sources, 342, pp. 17–23. doi.org/10.1016/j.jpowsour.2016.12.032.
- Wang, S., et al. (2019) Macrolevel Traffic Crash Analysis: A Spatial Econometric Model Approach. Mathematical Problems in Engineering, 2019, p. 5306247. doi.org/10.1155/2019/5306247.